20240807-004: WPD EII Quality Assurance Project Plan Part 2 — original pdf
Backup
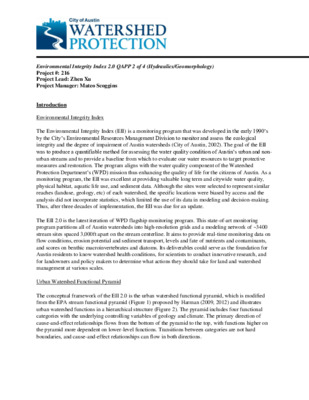
Environmental Integrity Index 2.0 QAPP 2 of 4 (Hydraulics/Geomorphology) Project #: 216 Project Lead: Zhen Xu Project Manager: Mateo Scoggins Introduction Environmental Integrity Index The Environmental Integrity Index (EII) is a monitoring program that was developed in the early 1990’s by the City’s Environmental Resources Management Division to monitor and assess the ecological integrity and the degree of impairment of Austin watersheds (City of Austin, 2002). The goal of the EII was to produce a quantifiable method for assessing the water quality condition of Austin’s urban and non- urban streams and to provide a baseline from which to evaluate our water resources to target protective measures and restoration. The program aligns with the water quality component of the Watershed Protection Department’s (WPD) mission thus enhancing the quality of life for the citizens of Austin. As a monitoring program, the EII was excellent at providing valuable long term and citywide water quality, physical habitat, aquatic life use, and sediment data. Although the sites were selected to represent similar reaches (landuse, geology, etc) of each watershed, the specific locations were biased by access and the analysis did not incorporate statistics, which limited the use of its data in modeling and decision-making. Thus, after three decades of implementation, the EII was due for an update. The EII 2.0 is the latest iteration of WPD flagship monitoring program. This state-of-art monitoring program partitions all of Austin watersheds into high-resolution grids and a modeling network of ~3400 stream sites spaced 3,000ft apart on the stream centerline. It aims to provide real-time monitoring data on flow conditions, erosion potential and sediment transport, levels and fate of nutrients and contaminants, and scores on benthic macroinvertebrates and diatoms. Its deliverables could serve as the foundation for Austin residents to know watershed health conditions, for scientists to conduct innovative research, and for landowners and policy makers to determine what actions they should take for land and watershed management at various scales. Urban Watershed Functional Pyramid The conceptual framework of the EII 2.0 is the urban watershed functional pyramid, which is modified from the EPA stream functional pyramid (Figure 1) proposed by Harman (2009, 2012) and illustrates urban watershed functions in a hierarchical structure (Figure 2). The pyramid includes four functional categories with the underlying controlling variables of geology and climate. The primary direction of cause-and-effect relationships flows from the bottom of the pyramid to the top, with functions higher on the pyramid more dependent on lower-level functions. Transitions between categories are not hard boundaries, and cause-and-effect relationships can flow in both directions. Figure 1. Stream Functions Pyramid (Harman et al. 2012). A framework for approaching stream assessment. Figure 2. EII 2.0 modified watershed functional pyramid. Each layer in this pyramid has a distinct modeling and monitoring approach. This implicitly includes geology as the underlying and fundamental framework for this pyramid, and the climate around it which drives the hydrologic cycle and many other factors that affect all layers. Hydraulics and geomorphology functions This QAPP focuses on the second category for hydraulics and geomorphology functions. Hydraulics functions transport water in the channel, on the floodplain and through sediments, whereas geomorphology functions transport sediment to create diverse bed forms and dynamic equilibrium. The energy associated with moving water has the ability to drive many important aspects of stream morphology and dynamics (Flores et al., 2006; Jain et al., 2006; Barker et al., 2009; Pike et al., 2010), making hydraulics functions closely related to geomorphology functions with many interrelationships between them. Harman (2012) outlines many parameters to consider in characterizing stream hydrology, hydraulics, and geomorphology. In all, there are 11 parameters suggested for assessment using 42 different measurement methods included in just the hydraulics and geomorphology portions of the stream functional pyramid. Of these, flow dynamics, as represented by stream power, and sediment transport capacity, as represented by modeled sediment discharge were selected for further evaluation. Stream power will be estimated from a GIS analysis using the 2021 Digitation Elevation Model (DEM) available for City of Austin (COA), combined with regression equations from local data on hydraulic geometry. Sediment discharge and concentration will be estimated from the Gridded Surface Subsurface Hydrologic Analysis (GSSHA) model output calibrated from local data. Estimation of hydraulic properties from regional regression equations Several of the hydraulic and geomorphic parameters suggested for evaluation are based on regional curves or equations for relating bankfull discharge and channel dimensions (i.e., width, depth and cross- sectional area) to drainage area. Determining bankfull discharge has additional benefits of predicting stable channel forms and equilibrium sediment transport. Although a 1.5 year recurrence interval discharge has been used as bankfull conditions in some studies, field data should be obtained on visual indicators of bankfull hydraulic geometry whenever possible (Harman 2012) and crucially before any design criteria are chosen. As a screening estimate of hydraulic geometry for the EII 2.0, we considered the available regional equations for Texas ecoregions available in Asquith (2020). We also considered regression equations that were developed from previous USGS work (Slade et al., 1996) and City of Austin (Olinde et al., 2021). In developing regional curves for USGS gages in Texas, Asquith developed and screened the datasets and performed linear regressions using logarithmic transformations of both the response and predictor variables. Response variables were the hydraulic properties of cross section area, top width, flowrate, depth, and velocity at estimated bankfull conditions. Predictor variables were watershed area (W), dimensionless main channel slope (S), and annual average precipitation (P). Two sets of equations were developed distinguished by the choice of data employed and treatment of ecoregion. The aggregate regional regression equations are calculated from all statewide measurements and ecoregion is treated as a categorical variable (“all regions”). The “region specific” equations are calculated where only measurements made at gages within each ecoregion are used in the regressions. In addition, regressions were performed for one (watershed area), two (watershed area and precipitation) or all three (watershed area, slope, and precipitation) of the predictor variables in the region-specific equations. All the equations contain a Duan retransformation bias correction factor (Helsel and Hirsch, 2002) applied to the left side of the equation. Using this factor in retransformation gives a median estimate of the hydraulic property rather than the biased arithmetic mean. Applicability of regional regression equations to small watersheds Ideally, the predictor variable range would be within the ranges used in the regressions; however, we are trying to estimate hydraulic properties of flow and bankfull top width at the entire range of points from headwaters above 320 acres to mouths of Austin creeks for use in stream power and sediment transport calculations (Harman, 2012; Van et al., 2021). Therefore, 48% of the areas for the EII 2.0 monitoring points will be below the minimum used in developing the equations, less than 2.31 sq mi. The regressions would strictly be valid only for those points within the ranges of the USGS gages used. Summary statistics for the two ecoregions of interest in the Austin area are included in Table 1. Table 1. Summary statistics for Blackland Prairie and Edwards Plateau ecoregions USGS gages in Texas. Asquith (2020) developed a third set of regression equations by subsetting the data for the range of USGS gages in the 2-120 sq mi contributing drainage areas. Considering the diagnostics of these regressions, they were not recommended for watersheds below 10 sq mi in contributing drainage area. For the 3,000 ft interval stream points in EII 2.0, we will have watersheds as low as 320 ac (0.5 sq mi) in drainage area up to Austin’s largest watershed, Onion Creek, with a contributing drainage area of 344 sq mi. Bankfull width from local data An analysis was also performed in 2021 using sites local to Austin areas watersheds and bankfull width estimates from field indicators. These data were collected as part of previous EII surveys (Clamann et al., 2019) and Stream Restoration Program studies (Adlong, 2015) as reported in Olinde et al., 2021. Figure 3 shows results of power law regressions from these studies along with additional Blackland prairie sites evaluated in Olinde et al., 2021. Figure 3. Bankfull width regressions with drainage area from Olinde et al., 2021 for Austin area data. Although the r-squared of regressions are relatively low, this could be expected from field indicators of bankfull width that are mainly visual in nature and the range of development in these watersheds. These low r-squared values are also reflected in the analysis of ecoregion USGS gage data in Asquith, 2020. Selected bankfull width equation The regression equations above were combined with those obtained from Asquith, 2020 in Figure 3 over the range of drainage acres we would consider in the EII 2.0. The local data regressions in general would predict lower widths for the same drainage area. The USGS regressions using the small watershed dataset showed a larger slope with the lower values closer to those predicted using just the local data. The highest values predicted were those for the entire range of gaged ecoregion watersheds. For the first estimate of stream power, the regressions from local data will be used as follows: Blackland Prairie: BFW(ft) = 3.95 × A0.19 (1) Transition: BFW(ft) = 5.96 × A0.20 (2) Edwards Plateau: BFW(ft) = 4.23 × A0.23 (3) Where BFW is the bank full width in ft. A is the contributing catchment area to the point in the stream in acres. The first estimates will use the transition zone regression (equation 2) for the entire set of EII 2.0 points and upgraded to the specific equations for each ecoregion area later. Ultimately BFW could be established through GSSHA for model runs at channel forming flows using natural cross sections approximating those measured in the field rather than trapezoidal channels. Figure 4. Bankfull width regressions from Asquith, 2020 combined with those from Olinde et al., 2021 for comparison. Channel forming flow estimates The other value required for calculation of stream power is the channel forming flow. This is typically taken as the average flow at bankfull conditions. Therefore, they are related to the regressions discussed above. However, average flows at bankfull conditions are only available at gaged locations. As mentioned above, bankfull conditions are often estimated by a low recurrence interval storm such as the 2-year or 1.5 year storm. This is a necessary simplification necessary to estimate stream power under stable channel conditions. A 1996 study by USGS divided the state of Texas into hydrologic regions and evaluated the peak flows for multiple recurrence interval storms based on watershed characteristics using gaged flow data. The 2-year flow equations for Region 5 including much of the Austin area was as follows: Q2 = 159 × A 0.680 (4) where Q2 is the 2-year recurrence interval discharge in cfs, and A is the contributing drainage area in square miles. Erosion and sediment routing The need to include geomorphology as a central component of watershed monitoring is recognized by the concept of hydromorphology (Orr et al., 2008; Vaughan et al., 2009). Specifically, fluvial geomorphology connects stream power to the physical shapes of rivers via the water and sediment transport processes and the landforms they create. Whenever a fluvial feature changes form, there is a local imbalance in the movement of sediment to and from the site. An understanding of how a river system collects, transports and deposits sediment is therefore central to addressing both applied and theoretical questions regarding how changes in catchment conditions affect channels, how long the effects will last and what the sequence of responses will be. Sediment budgets are tools for building that understanding, which define the most fundamental aspect of landform evolution: mass conservation. Information about whether the sediment budget of a particular fluvial landform or a fluvial system is in a steady state is as basic a descriptor as the mean annual flood, mean annual runoff or other commonly used characterizations of fluvial systems, which calls for the need to quantify sediment supplies and transport rates. Geomorphologists and engineers spent the last century developing empirical and process-based equations for modeling sediment transport in rivers, the proliferation of which has been criticized as excessive – ‘there appears to be more bed load formulae than there are reliable data sets by which to test them’ (Gomez and Church, 1989). Many sediment transport equations rely on shear stress (requiring flow depth) (Meyer-Peter and Müller, 1948; Parker, 1990), velocity (Brownlie, 1982), or both (Engelund and Hansen, 1967), which are physically linked to sediment transport processes but hard to measure as a result of their temporal and spatial variability. Some researchers also suggested approaches utilizing stream power (Reinfelds et al., 2003; Phillips and Desloges, 2014), which can be estimated in a relatively straightforward way by channel slope and width (e.g., by remote sensing) and discharge data (e.g., by stream gages, hydrologic models, or regional empirical relationships). Nowadays different sediment transport equations are often considered part of a toolbox, where each tool has its own purpose. It is important to choose the right ones according to the needs of end-users. Erosion and fluvial sediment transport in the EII 2.0 will be estimated by the Soil Routing module of GSSHA, which is an updated version of the CASCade two-dimensional SEDiment model (Johnson et al., 2010). GSSHA is a fully distributed and physically based model developed by the U.S. Department of Defense (Downer and Ogden, 2004). The model employs a mass-conserving solution of partial differential equations to produce the different components of hydrologic processes. As compared to CASC2D, GSSHA replaces the Green-Ampt infiltration equation in the runoff estimates with three alternatives: the three-layer Green-Ampt, the Green-Ampt infiltration with redistribution, and the 1-D formulation of the Richards’ equation. For sediment concentration estimation, GSSHA substitutes explicit finite difference of diffusive waves for explicit two-step finite volume schemes to convey water for both 1-D channel flow and 2-D surface runoff. The GSSHA model also adds new features/equations including the 2-D saturated groundwater flow computation, Deardorff or Penman–Monteith evapotranspiration methods, and Thiessen polygon interpolation method (Downer et al., 2008). Nowadays GSSHA has been successfully applied in a variety of research, civil engineering, and military contexts (Zhang et al., 2023). Project History The EII is a comprehensive biological, chemical and physical monitoring tool designed to assess the ecological integrity and the comparative degree of impairment of Austin’s watersheds. It was originally developed and tested in the urban watersheds in 1994 and 1995 and initiated citywide in 1996. The methods of the original program were documented in Environmental Integrity Index Methodology (COA 1999). By the year 2000, water quality sampling was conducted quarterly, and the biological and habitat surveys were completed annually. Fifty of the City of Austin planning watersheds were grouped into three phases with approximately 150 total sites sampled on a three-year rotating basis (~50 sites sampled per year). Phase 1 primarily included the urban watersheds sampled historically under the Water Watchdog volunteer program (Project 20) while Phase 2 and Phase 3 included suburban and developing watersheds. In 2009, the watersheds were regrouped into two phases for sampling on a two-year rotating schedule. This regrouping was designed to increase site visit frequency to improve the resolution of temporal trend evaluation and align with frequency requirements of the Clean Rivers Program of the Texas Commission on Environmental Quality. Although the methods have remained relatively stable over time, the project has evolved slowly due to advancements in technology, staff bandwidth, and state-of-the-science. A retrospective of the project after 25+ years of work indicated that much could be gained with strategic advancements to the program. The EII has been used in the Department’s Master Plan process to evaluate the current water quality conditions of Austin’s watershed. Additionally, it has been used to satisfy a portion of the City’s National Pollution Discharge Elimination System (NPDES) permit. Perhaps the most valuable product of the EII is a long-term dataset that substantiates a baseline condition from which models can be developed. Objective Zero, a concept which WPD uses to identify target conditions for aspirational environmental goals (Herrington, 2017), could not be efficiently informed by the structure of EII as it existed in 2022. The 2022 fiscal year was the last year of traditional phase-based quarterly water quality sampling. Field and analysis methods were developed through four subcommittees: Hydrology, Geomorphology, Physicochemical and Biology. Each subcommittee evaluated the successes and failures of the past 30 years of EII program, reviewed the relevant body of work (both COA projects and literature), and discussed goals and aspirations to meet the needs (both current and future) of the rapidly growing community in the context of climate change and evolving environmental stressors. Related Reports and Publications • Stream Corridors in The Blackland Prairie RR-21-01 • Erosion Mitigation Design Manual, Preliminary Results: Training Watershed DR-19-05 • Developing A Stream Stability Index SR-15-09 • Qc threshold departs from theoretical critical discharge in urban watersheds: the role of streambed mobility data in managing the urban disturbance regime Freshw. Sci. 41:3 • Erosion Hazard Zone Mapping and Geomorphic Assessment of Eastern Watersheds HDR- • Technical Procedures Manual for Watershed Erosion Assessments: Final Report C.I.P. NO. 485- 00310·106328 617-2000 Abstracts for Related Reports and Publications • RR-21-01 (Olinde et al., 2021): This study conducted vegetation and channel stability assessments along Blackland Prairie streams in east Austin. Results show that both of woody riparian vegetation cover and diversity and the ecological health and function of the riparian buffers are relatively low at the studied sites as compared to those in the western Austin’s Edwards Plateau and central Austin’s transition area. The clearing of the historical riparian forests likely increased the vulnerability of the fine-grained Blackland Prairie streams to incision and instability, and the scarcity of seed sources for riparian vegetation across these eastern watersheds likely extends lag times for recovery. The study highlighted the environmental risks in the Blackland Prairie and outlined several existing management strategies that could be updated with its findings. • DR-19-05 (Hawley et al., 2020): This report summarizes data collected from the headwaters catchment of the Country Club West (CCW) watershed in Austin, Texas, as preliminary monitoring and modeling efforts to develop an internal City of Austin Watershed Protection Department (WPD) design manual for watershed-scale erosion mitigation. It models Stormwater Control Measure (SCM) scenarios and quantified excess erosive power for each modeled wet weather event as the amount of sediment transport capacity that exceeds undeveloped conditions. The initial sediment mobility data from several events in the studied period (May 30 – June 30, 2019) suggested that the local erosive threshold for the streams in the catchment corresponds to a critical Shields number of approximately 0.047. The initial results also suggested that the reduction of excess erosive power for a 1-year event in the catchment varies depending on the SCM scenario, ranging from 0% to 100%. • SR-15-09 (Davis et al., 2015): This study assessed geomorphic, hydrologic, and riparian characteristics in Austin watersheds to determine effective indicators of stream channel instability. A total of 15 long-term routine monitoring parameters were evaluated at 21 stream reaches for the development of a Stream Stability Index. Results showed that that Scouring and Deposition was the best performing parameter classifying all site locations, whereas some other parameters also worked for specific ecoregions. • A peer-reviewed paper published in Freshwater Science (Hawley et al., 2022) used data collected from Austin watersheds and two other regions with hydrogeomorphically distinct settings to investigate variability in threshold discharge (Qc). This study showed how both high- and low- tech monitoring protocols could be used to constrain a Qc estimate, depending on monitoring program goals and budgets. The authors suggested conducting streambed mobility monitoring to calibrate and validate Qc estimates for a stream or region prior to making large investments in stormwater interventions. Professionals from the City of Austin Watershed Protection Department participated in data collection and manuscript preparation. • HDR-00310·106328 (HDR, 2011): The City of Austin consulted HDR Engineering, Inc. (HDR) in 2011 to perform fluvial geomorphic assessments and establish setbacks areas of potential channel erosion for streams before significant development at the Gilleland, Harris Branch, Decker and Elm watersheds along the eastern potions of the City. Detailed fluvial geomorphic assessments were completed for approximately 75 geomorphic like reaches to evaluate historical stream planform and profiles, document current stream conditions, and predict channel response to future development. Erosion hazard zone (EHZ) delineations were produced for streams with associated drainage areas of 64 acres or more. Results showed that the eastern watersheds exhibited an overall state of stability, and the focus should be on preserving the natural dynamic geomorphic qualities of these streams by regulating development within the EHZs defined. • C.I.P. NO. 485-617-2000 (RC&A, 1997): The City of Austin hired Raymond Chan and Associates, Inc. (RC&A) in 1997 to prepare a technical procedures manual as a guideline for the completion of the watershed erosion assessments for seventeen watersheds. The report indicated that urbanization resulted in a fundamental shift in the flow regime due to the decrease in hydrologic storage (abstractions) and the decrease in basin response time. That lead to a significant increase in the frequent, or minor system flow events which controlled the morphology of the active channel in streams in the City of Austin. The associated increase in instream erosion potential results in significant enlargement of the stream channel. The channel would preferentially widen until the erosive power of the prevailing flows was insufficient to erode the banks. The report concluded that these observations showed an ongoing 'valley formation' process, which was evident in many of the older developed watersheds within the City of Austin. Project Objective The objectives of this study are threefold: tools. 1. Calculate slopes and stream power using existing digital elevation model (DEM) data and GIS 2. Collect water samples from spatially representative sites of Austin watersheds to calibrate the Soil Erosion and Sediment Routing module of GSSHA (Gridded Surface Subsurface Hydrologic Analysis) model. 3. Characterize the relationship between sediment transport and river hydrology (i.e., sedograph) for Austin watersheds, and discern the impact of stream power on sediment loads (in mass per time) for all EII 2.0 stream network nodes on an annual basis. Policy Relation Results from the EII 2.0 monitoring program will be used as an environmental input to the Watershed Health Decision Support System (WHDSS). This system is a part of the Department’s Strategic Plan in prioritizing its work in either improving or preserving stream conditions throughout the City, while incorporating equity. Specifically, through WHDSS, the EII will identify those streams that require environmental mitigation or protection (or some combination of both). Specifically for the stream power and sediment transport data derived from this QAPP: 1. The City of Austin has an Erosion Hazard Zone ordinance which provides geomorphic buffers along streams. They are very general and rely on the development review process for implementation. Providing estimates of stream power and sediment flux though out our stream network will be an excellent complement to the Erosion Hazard Zone ordinance and potentially provide the framework for improved erosion development policies moving forward. 2. The current project prioritization for erosion and channel rehabilitation does not include any predictive tools, but rather relies completely on an inventory of observations and complaints of known erosion problems (mostly bank erosion and threats to structures). The framework being provided by this QAPP (and integrated into the WHDSS) will bring powerful planning level tools to more effectively and efficiently manage watershed-scale drivers of channel dynamics and provide optimized solutions instead of a reactive patchwork of repairs. Project Committee The EII 2.0 project has four separate QAPP committees structured in accordance with the modified functional pyramid: Hydrology, Hydraulics/ Geomorphology, Physicochemical, and Biology. The hydraulics/ geomorphology committee structure (ARCI) is detailed in Table 2. Table 2. EII 2.0 Hydraulics/ Geomorphology Committee Role Personnel Planning Analysis Modeling Project Manager Mateo Scoggins Project Lead Zhen Xu Data Manager Robert Clayton Data Collection C A R A R C I A I I A I Report Writing A R I GIS Leader Committee Member (DA/DS) Committee Member (DA/DS) Committee Member (GA) Committee Member (GA) Committee Member (ASC) Committee Member (Other subcommittees) Yazmin Avila Ed Peacock Abel Porras Courtney Guidry William Burdick Scott Hiers Angel Santiago, Andrew Clamann, Christina Bryant R R R C C C C R R C C I C I R R R C I I I C R R I I I I R R C I I I C * A = Accountable (ensures project has needed resources, held accountable if not completed); R = Responsible (leads development of study components, performs and delegates tasks, reviews deliverables before deemed complete); C = Consulted (specialist in specific component of study, available for comments); I = Informed (kept in the loop, comments optional) Table 3. Analysis Laboratory Lab USGS Kentucky Water Sediment Laboratory • Suspended sediment concentration analysis: Annual Cost $28,008 sample o Non-tared (weighed) bottles, $9 per bottle o One additional bottle to make composite, $9 per bottle • Number of samples for FY 24: o Suspended-sediment sand-fine break (includes concentration)/Filtration method, $59 per o 9 monitoring sites o 2 discrete sampling events with 14 samples per event o 4 composite sampling events with 1 sample per event (please see sampling schedule below for details) • Total budget estimate for FY 24: o Discrete samples: ▪ w/o bottle weighing by COA: 9 × 2 × 14 × ($59 + $9) = $17,136 ▪ with bottle weighing by COA: 9 × 2 × 14 × $59 = $14.868 o Composite samples: ▪ w/o bottle weighing by COA: 9 × 4 × ($59 + 13 × $9 + 14 × $9) = $10,872 ▪ with bottle weighing by COA: 9 × 4 × ($59 + 13 × $9) = $6,336 o Total: ▪ $28,008 w/o bottle weighing by COA ▪ $21,204 with bottle weighing by COA Personnel Time Table 4. Estimated Time Budget Task Personnel Work Time Project management Zhen Xu Estimated 80 hrs annually Field Work GIS Analysis AWR staff Estimated 250 hrs annually DA/DS, GA staff Estimated 120 hrs annually Data Entry/QC AWR, DA/DS staff Estimated 160 hrs annually Data management AWR, DA/DS staff Estimated 160 hrs annually AWR, DA/DS staff Estimated 500 hrs annually AWR staff Estimated 40 hrs annually Modeling Reporting Project Funding Table 5. Project Funding Sources FDU (Fund Department Unit) 5100 6300 3135 (Lab, Analysis) 5100 6300 3140 (Field) Project Schedule Table 6. Project Schedule Target FY24-Q1 FY24-Q2 FY24-Q3 FY24-Q4 FY25-Q1 Oct-2024 FY25-Q1 Nov-2024 FY25-Q1 Dec-2024 2 storm events (one ≥ 1″ rain and the other < 1″ rain) Discrete sample collection Composite sample collection Field data QA/QC Stream power estimate update Stream power validation Data analysis/modeling Annual report drafting Annual report review ✓ ✓ ✓ Aligned with EII 2.0 hydrograph generations ✓ ✓ ✓ ✓ ✓ ✓ ✓ ✓ ✓ ✓ ✓ ✓ ✓ Experimental Methods Sampling locations Candidate monitoring sites were selected by hierarchical cluster analysis, which seeks to partition all identified nodes in Austin watersheds into distinct groups with similar observations. The cluster analysis used the following watershed characteristics: • Soil Survey Geographic Database (SSURGO) soil infiltration data (i.e., total, mean and standard deviation of infiltration volume) • Annual total nitrogen and phosphorus loading • Erosive potential by slopes (areal slope for drainage area, areal slope of buffered creek, channel slope) ✓ ✓ • Percent impervious cover • Watershed catchment size Cluster analysis identified several candidate sites that were the most representative of each cluster. The project team completed field evaluations at the sites to determine suitability for access and installation of monitoring station. One site for each cluster was selected in addition to one additional site to monitor a stream that was influenced by treated wastewater effluent. Table 7. Continuous Monitoring Site Locations Watershed Site ID Site Short Name Cluster ID BAR BUL ELM GIL ONI RIN SLA WLN WLS 51 Barton @ Lost Creek Blvd 142 Bull @ Spicewood Springs Rd 7th Crossing 2956 Onion @ Ped Xing south of William Cannon 3068 Elm @ FM969 1192 Gilleland @ FM 973 233 Rinard @ Bradshaw Rd 623 Slaughter @ FM1826 5285 Walnut @ Metric Blvd 5* 2 4 5 8 1 3 6 7 463 Wells Branch @ Walnut Creek Park Rd * Although site 1192 is located within one of the eight clusters of the model, this site has been selected to characterize stream segments that are wastewater effluent driven. Figure 5. Locations of EII 2.0 monitoring sites The preference is to input the new EII 2.0 sites to existing sites in the WRM database, so new data can be compared to historic data. All EII 2.0 sites will be inputted as the nearest existing EII 1.0 site unless a site does not exist at that location or exceeds a reasonable distance (~50 feet) to be listed as the same site. If this occurs a new site will be added to the database. Note that since site equipment must be mounted near solid structures, in some instances this tolerance was exceeded. One shelter will be constructed at each monitoring site to protect equipment (e.g., ISCO samplers, batteries, control boards, etc). Shelters are located out of the floodplain to reduce the chance of inundation and thus protect components, but within the maximum tubing length of the autosampler pump (100 feet), and the maximum head of the autosampler pump (28 feet in elevation). Data collection methods An ISCO Signature® flow meter will be installed for open channel flow measurements. At each monitoring site, the flow meter will measure stage utilizing one or more of the following methods: bubbler level, ultrasonic elevation, or laser level with velocity readings. The stage measured by the flow meter will be used to signify rainfall-runoff events and will trigger the autosamplers to enable and begin collecting stormwater samples. Additional detail on the stage sensor is available in the EII 2.0 Hydrology QAPP. An ISCO 3700 autosampler will be installed at each sampling site for water sample collection. The ISCO Signature® flow meter controls the autosampler to enable it and control pacing. When a stage threshold is triggered, the autosampler will activate and collect the sample per advance programming. A maximum of 14 discrete samples per event will be collected by the sampler and delivered to the analytical lab after each event. The equipment listed here is specifically for this QAPP. Additional details on hydrology equipment (e.g., ISCO Tipping Bucket Rain gage) and water quality equipment (e.g., BLZZRD™ portable autosampler and data sondes), are available in the EII 2.0 Hydrology and EII 2.0 Physicochemical QAPPs, respectively. Sample data collected during the initial years will be primarily used for model calibration. The QAPP committee will regularly assess progress. Once the model calibration aligns with the committee's standards, the QAPP will undergo revisions to incorporate new elements for a validation phase. Parameters All water samples collected by the ISCO 3700 autosampler will be directly delivered to the USGS Kentucky Sediment Laboratory for suspended-sediment sand-fine break and concentrations using ASTM’s suspended sediment concentration (SSC) method D3977-97 (ASTM, 1997), which specify the use of whole original water samples without subsampling. No mandatory sample preservation is needed, and the holding time is 120 days. Sampling schedule The annual goal is to collect samples from six storm events for the calibration needs of the Soil Erosion and Sediment Routing module of GSSHA model. • Two Storm events will be Discreet sampling. Each discreet sampling event will collect up to 14 discrete samples per BLZZRD™ portable refrigerated autosampler. o For 1 event, samples will be collected with precipitation greater than 1 inch. o For 1 event, samples will be collected with precipitation less than 1 inch. • Four events will be collected following TCEQ seasonal monitoring periods or composite samples. Each monitoring site will collect up to 14 aliquots that will be composited into one sample. o Season 1 (Wet): September – October o Season 2 (Dry): November – February • All sites will not necessarily have samples collected during the same event. If a season is missed, o Season 3 (Wet): March – June o Season 4 (Dry): July - August it will be resampled the following year. AWR automated stormwater samples will be time weighted. • 15-minute intervals • Total of 3.5 hours USGS automated sampling will be flow weighted. QC specifications Sample collection and preservation will be completed in accordance with WPD Standard Operating Procedures (SOPs, WPD Applied Watershed Research Section - Storm_SOP_October_2020.pdf - All Documents (sharepoint.com)). • Pre-Storm Event: When a qualifying rain event is anticipated, clean 950 mL bottles will be placed in the ISCO 3700 autosamplers at each site. • Post-Storm Event: After a qualifying rain event and when conditions are safe to do so, the bottles will be collected from the autosamplers at each site within one week from the last aliquot collection time. Bottles will be capped with clean lids and placed on ice and cooled to <2 °C for transport to USGS Kentucky Sediment Laboratory for analysis. • Sample volume of the autosamplers will be checked and adjusted on a quarterly basis. • WPD staff will complete the chain of custody (COC) and conduct data validation for each sampling event (Stormwater Monitoring Workflow.docx (sharepoint.com)). Personnel collecting samples from autosamplers for laboratory analysis will generate a COC utilizing the Water Resources Monitoring (WRM) database prior to sample collection. o Each monitoring site and sampling event will have a unique COC with site number, name and description, sample type (e.g., composited or discrete), sample ID number, sample date, and sample time. o Laboratory personnel and COA scientists will sign and date the COC documenting the relinquish and acceptance of sample custody (WRM User Guide (sharepoint.com)). o The COC will be printed and delivered to the lab along with the water quality samples. Analytical Methods Stream Power Total stream power per unit channel length (Ω, in W m-1) can be mathematically defined as: Ω = γ × Q × Se (5) where γ is the specific weight of water (9810 N), Q is discharge (m3 s−1) and Se is energy slope (m m−1), which is approximated by the water‐surface slope (S) (Knighton, 1999; Barker et al., 2009). By incorporating channel width into the equation, stream power can then be used to refer to the potential energy supplied to a unit area of the channel bed (Barker et al., 2009). Specific stream power (ω) can be defined as: ω = Ω / wd (6) where wd is the channel width in meters. Specific stream power has units of W m−2. Initial stream power estimations were completed using the equation above and parameters derived from the algorithms described in the introduction (i.e., equation 4 for discharge estimations, equation 2 for bankfull width estimations, and GIS analysis for other parameters). These initial stream power estimates will be updated annually using peak flows from current EII generated hydrographs. Validation of stream power will occur by comparing incidents of erosion from 311 complaints. GSSHA Erosion and Sediment Routing GSSHA simulates erosion and sediment routing by linking two-dimensional (2-D) overland sediment erosion to one-dimensional (1-D) transport in a stream channel. 1. The model firstly calculates particle detachment by raindrops and surface runoff. a. Detachment by raindrops is considered to be a function of rainfall momentum, which is also a function of rainfall intensity. DR = K1 × CW × CG ×Ci × MR (7) where DR is the detachment capacity rate (kg m-2 s-1), K1 is the soil erodibility factor for detachment by raindrop impact (J-1), CW is the water depth correction factor, CG is the canopy cover factor, Ci is the cover-management factor, and MR is the moment squared for rainfall ((kg m s-1)2 m-2 s-1). b. Surface runoff detaches soil particles by exerting a shear stress that breaks the bonds between particles. The detachment capacity rate by surface runoff is estimated by: DC = a × (τ – τcr)b × (1 - ) (8) 𝐺 𝑇𝑐 where DC is the detachment capacity rate (kg m-2 s-1), a and b are empirical coefficients, τ is the flow shear stress (Pa), τcr is the critical shear stress, G is the sediment load (kg m-2 s-1), and Tc is the sediment transport capacity of surface runoff (kg m-2 s-1). 2. GSSHA then calculates the transport capacity of surface runoff using one of the manually selected transport equations for 2-D overland flow transport. a. Kilinc Richardson qs = 25.5 × q2.035 × Sf 1.664 × (9) 𝐾 0.15 where qs is the sediment unit discharge (kg m-1 s-1), the factor 25.5 is an empirical constant, q is unit discharge (m2 s-1), Sf is the friction slope (m m-1), and K is a combined factor (0-1) that consists of the combined influence of soil erodibility, vegetation, and land-use. b. Engelund-Hansen 0.05×𝐵×𝑉2×ℎ 3 2×𝑆 (𝑠−1)2×𝐷𝑖×√𝑔 3 2 Gi = K × Fi × (10) where Gi is the volumetric sediment transport rate of ith size fraction (m3 s-1), K is the calibration coefficient (=1 for standard equation), Fi is the proportion of ith faction in the parent material or deposited layer, B is the width of flow (m), V is the mean water velocity (m s-1), h is the flow depth (m), S is the water surface slope, s is the specific gravity of ith fraction, Di is the mean size of ith fraction (m) and g is the gravitational acceleration (m s-2), . The factor 0.05 in the equation was developed from empirical data. c. Unit stream power qs = 0.316 × (𝑆𝑓 × 𝑉𝑒𝑙)2.59 × 𝐷50 where qs is the sediment unit discharge (kg m-1 s-1), the factor 0.316 is an empirical constant, Sf is the friction slope (m m-1), Vel is velocity (m s-1), D50 is the grain size parameter (µm). −0.39 (11) d. Effective stream power 1.5 (𝜌𝑔𝑞𝑆𝑓) 2 3 𝑅 qs = 4.6 × 10-7× [ ]1.75 × 𝐷50 −0.56 (12) where qs is the sediment unit discharge (kg m-1 s-1), ρ is density of water at 20 °C (kg m3), g is the gravitational acceleration (m s-2), q is unit discharge (m2 s-1), Sf is the friction slope (m m-1), R is flow depth (m), D50 is the grain size parameter (µm) e. Slope and unit discharge 𝐵 × 𝑞𝑐 (13) qs = A × 𝑆𝑓 where qs is the sediment unit discharge (kg m-1 s-1), Sf is the friction slope (m m-1), A, B and C are empirical constants. i. If D50 < 33.0, A = 6.16595, B = 1.9, C = 1.1. ii. If 33.0 ≤ D50 < 61.0. A = 10.964, B = 1.94, C = 1.1. iii. If 61.0 ≤ D50 < 122.0, A = 9.322, B = 1.77, C = 1.79. iv. If 122.0 ≤ D50 < 190.0, A = 64.565, B = 2.96, C = 2.18. v. If D50 > 190.0, A = 10.964, B = 1.94, C = 1.1, where D50 is the grain size parameter (µm). f. Transport capacity by shear velocity qs = A × (µ - B)C (14) where qs is the sediment unit discharge (kg m-1 s-1), µ is shear velocity (m s-1), A, B and C are empirical constants. i. If D50 < 33.0 and µ > 1.4, A = 0.035, B = 1.40, C = 2.88. ii. If 33.0 ≤ D50 < 61.0 and µ > 1.4, A = 0.052, B = 1.40, C = 2.95. iii. If 61.0 ≤ D50 < 122.0 and µ > 1.45, A = 0.029, B = 1.45, C = 3.74. iv. If 122.0 ≤ D50 < 190.0 and µ > 1.55, A = 0.024, B = 1.40, C = 4.14. v. If µ > 1.8, A = 0.0092, B = 1.80, C = 5.06, 3. GSSHA specifies 1-D channel routing of sediments by where D50 is the grain size parameter (µm). a. employing the unit stream power method for routing user-specified sand-size total-load in stream channels: Φ(Cs, USo, U*, ν, w, d) = 0 (15) where Cs is the total concentration of sand-size sediment particles in motion, USo is unit stream power (Length Time-1), U* is the shear velocity (Length Time-1), ν is kinematic viscosity of the sediment-water mixture (Length Time-2), w is fall velocity of the sediment (Length Time-1), d is particle diameter (Length), and b. employing the general 1-D advection-dispersion equation for particles smaller than the user-specified value of sand. Those particles are assumed to be in suspension and transported as wash load following: - + (𝐷 𝜕 𝜕𝑥 𝜕𝑀 𝜕𝑥 𝜕(µ𝑀) 𝜕𝑥 ) + K × M = S (16) 𝜕(𝑀) 𝜕𝑡 where µ is flow velocity in the x-direction (Mass Time-1), M is the mass of transported fine sediment which is equal to SSC concentration (Mass Length-3) multiplied by the volume (Length3), K is the decay coefficient (Time-1), and S is the term for all sources and sinks (Mass Time-1). 4. GSSHA outputs for erosion and sediment routing module are volumetric sediment flux (in m3 s-1) and suspended sediment concentration (in mg L-1). Field collected samples will be used to calibrate the suspended sediment concentration output (Figure 6, Sith and Nadaoka, 2017). Figure 6. An example of using field-collected sample suspended sediment concentrations (SSC) to calibrate GSSHA Erosion and Sediment Routing module outputs. The SSC range next to the y-axis is for illustration purposes only and is not made based on field observations. For GSSHA modeling, sediment data collected during the first few years will be primarily used for model calibration. The QAPP committee will continuously review the progress. Once model calibration performance meets the committee’s expectations, the QAPP will be updated for a validation phase with new contents potentially added for sampling locations, sampling schedule, laboratory costs and analytical methods. Impact of Stream Power on Sediment Loads Sediment loading can originate from two sources, upstream or upland. A relationship between stream power and sediment loads can be examined to discern the impact of upstream erosion on sediment loading. The annually updated stream power calculations will be paired with annual sediment loads (the area under the sedograph curve) calculated using WMS for each point. The results from this analysis will test the hypothesis that higher correlations between stream power and sediment loading indicate that the source of sediment is more likely from upstream erosion than upland. This can then point to potential solutions of mitigating any excessive sediment transport. Linkage of the geomorphology/hydraulics category to other categories in the EII 2.0 framework • Outputs from the EII 2.0 hydrology modeling (e.g., discharge, infiltration) will be used: o as input variables for stream power estimations and validation, as well as 1-D channel o routing of sediments. to calculate sediment loading and help characterize the relationship between sediment transport and river hydrology (i.e., sedograph). • Channel routing of sediments from this category and nutrients from EII 2.0 physicochemical modeling both use the 1-D advection-dispersion equation. According to the GSSHA user’s manual, the equation needs to be calibrated for sediment at first as part of the prerequisites for constituent modeling (e.g., nutrients). • Outputs from the geomorphology/hydraulics category (i.e., SSC) can potentially be used as input variables for the statistical model used in EII 2.0 biology modeling. Assumptions Stream Power The spatial resolution of a DEM can affect the outputs for elevation, slope, stream network, subwatershed and watershed area, hence a DEM selection is critical in the watershed delineation process. The most recent DEM data available for City of Austin is a 2ft resolution with more detailed terrain representation, covering the extent of COA and ETJ (extraterritorial jurisdiction) boundaries. We evaluated the performance of the 2ft DEM for watershed delineation using techniques and tools available in ArcGIS Pro 2.9.5. This approach in automated watershed delineation resulted in long processing times when delineating the watersheds on our testing area. It was time consuming when trying to run flow direction and flow accumulation pre-processes at the whole 76 watershed extent. The DEM was resampled at a 4ft resolution, with the assumption that the watershed areas would be similar (Figures 7-9). Figure 7. Stream network greater than 320 acres using 2ft DEM (a) and resampled 4ft DEM (b) Figure 8. Watersheds delineated by different DEMs, 2ft (a) and resampled 4ft DEM (b) Figure 9. Watershed areas in acres. GSSHA Erosion and Sediment Routing Assumptions 1. Detachment by surface runoff a. Within a grid cell, rill erosion and flow are assumed to be uniformly distributed. b. Detachment is assumed to be linearly proportional to the excess shear stress. 2. Sediment Transport in Channels of the channel. a. The degradation of trapezoidal channels is assumed to occur uniformly from the bottom b. Deposition is assumed to cover the entire bottom width of the channel, whether in the previously eroded bottom or in the trapezoidal section. c. To take the gravitational force into consideration, deposition is assumed to be proportional to the square of water depth, starting from the recorded maximum water surface elevation. d. Particles with sizes smaller than the user-specified value of sand are assumed to be in suspension upon entering the channel and are transported as wash load. e. Channels are neither a source nor a sink of fine sediments. Reporting An annual report will be prepared to summarize progress in data collection and whether model needs are met. A decision will be made then on whether sampling strategies need to be adjusted for the following sample year. Distribution The most recent QAPP is available in the WPD SharePoint. The project lead is responsible for informing all committee members via email if significant changes are made to the QAPP. Change Procedures The Project Lead will maintain this QAPP. Proper document management procedures are followed during any update and the QAPP will be locked for editing. The original approved QAPP will be kept as documentation and any changes to the are summarized in the QAPP Revision History below. QAPP Acceptance Subcommittee This QAPP and subsequent substantive changes shall be ratified by consensus of the QAPP Acceptance Subcommittee. Table 8. QAPP acceptance subcommittee Role Project Manager Project Lead (QAPP 2 of 4 Hydraulics/Geomorphology) Committee Member (DA/DS) DA/DS Supervisor Data Manager (DA/DS) GIS Lead Lead geologist Hydrology Project Lead (QAPP 1 of 4) Physicochemical Project Lead (QAPP 3 of 4) Biology Project Lead (QAPP 4 of 4) Name Mateo Scoggins Zhen Xu Ed Peacock Abel Porras Robert Clayton Yazmin Avila Scott Hiers Christina Bryant Angel Santiago Andrew Clamann Date of Acceptance 11/14/2023 (!) 11/13/2023 11/13/2023 11/13/2023 11/13/2023 11/14/2023 11/13/2023 11/09/2023 11/10/2023 11/13/2023 QAPP Revision History The date, contact name, and brief description for all substantive changes to this QAPP shall be documented in the Revision History (Table 9). Legislative markup or other details documenting the specific changes (as necessary) shall be added to Appendix A Change Log. Table 9. Revision History Date Modified By Modification Description Date of QAPP Acceptance Subcommittee approval References Adlong M. 2015. Enlargement and instability of stream channels in Austin, Texas: When to restore? In: Urban Riparian Symposium. Austin, TX. American Society for Testing and Materials (ASTM). 1997. Standard test methods for determining sediment concentration in water samples (ASTM Designation: D-3977-97), ASTM, West Conshohocken, Pennsylvania. Asquith, W.H., Slade, R.M.Jr. 1996. Regional equations for estimation of peak-streamflow frequency for natural basins in Texas. U.S. Geological Survey. Water Resource Investigations Report 96-4307. Austin, TX. Asquith, W.H., Gordon, J.D., and Wallace, D.S. 2020. Regional regression equations for estimation of four hydraulic properties of streams at approximate bankfull conditions for different ecoregions in Texas: U.S. Geological Survey Scientific Investigations Report 2020–5086, 45 p., https://doi.org/10.3133/sir20205086. Barker D.M., Lawler D.M., Knight D.W., Morris D.G., Davies H.N., Stewart E.J. 2009. Longitudinal distributions of river flood power: the combined automated flood, elevation and stream power (CAFES) methodology. Earth Surface Processes and Landforms 34: 280–290. Brownlie, W.R. 1982. Prediction of flow depth and sediment discharge in open channels. PhD Dissertation, California Institute of Technology. City of Austin, 2002. Environmental Integrity Index Methodology. Environmental Resources Management Division, Watershed Protection Department, City of Austin. Water Quality Report Series COA-ERM 1999-01. https://services.austintexas.gov/watershed_protection/publications/document.cfm?id=186267. Clamann A, Jackson T, Clayton R, Richter A. 2019. Environmental Integrity Index phase I & II (2015- 2016) watershed summary report. City of Austin. Davis, S., Richter, A., Scoggins, M. 2015. Developing a stream stability index. City of Austin Watershed Protection Department, SR-15-09. Downer, C.W., Ogden, F.L., Byrd, A.R. 2008. GSSHAWIKI User s Manual, Gridded Surface Subsurface Hydrologic Analysis version 4.0 for WMS 8.1. Engineer Research and Development Center, Vicksburg, Mississippi. Downer, C.W., Ogden, F.L. 2004 GSSHA: Model to simulate diverse stream flow producing processes. J. Hydrol. Eng. 9, 161–174. https://doi.org/10.1061/(ASCE)1084-0699(2004)9:3(161). Engelund, F., Hansen, E. 1967. A monograph on sediment transport in alluvial streams. Teknish Forlag Technical Press: Copenhagen, Denmark. Flores A.N., Bledsoe B.P., Cuhaciyan C.O., Wohl E.E. 2006. Channel‐reach morphology dependence on energy, scale, and hydroclimatic processes with implications for prediction using geospatial data. Water Resources Research 42(W06412): 15. https://doi.org/10.1029/2005WR004226. Gomez, B., Church, M. 1989. An assessment of bed load sediment transport formulae for gravel bed rivers. Water Resources Research 25: 1161–1186. https://doi.org/10.1029/WR025i006p01161. Harman, W.A. 2009. The functional lift pyramid (presentation). Mid-Atlantic Stream Restoration Conference, Morgantown, WV. Harman, W., Starr, R., Carter, M., Tweedy, K., Clemmons, M., Suggs, K., Miller, C. 2012. A function- based framework for stream assessment and restoration projects. US Environmental Protection Agency, Office of Wetlands, Oceans, and Watersheds, Washington, DC EPA 843-K-12-006 Hawley, R.J., Russell, K., Olinde, L. 2022. Qc threshold departs from theoretical critical discharge in urban watersheds: the role of streambed mobility data in managing the urban disturbance regime. Freshw. Sci. 41:3. https://www.journals.uchicago.edu/doi/10.1086/720939. Hawley, R.J., Korth, N., Acosta, S., Olinde, L. 2020. Erosion mitigation design manual, preliminary results: Training watershed DR-19-05. City of Austin Watershed Protection Department. HDR. 2011. Erosion Hazard Zone Mapping and Geomorphic Assessment of Eastern Watersheds. HDR Engineering, Inc., 130p. services.austintexas.gov/watershed_protection/publications/document.cfm?id=206383. Helsel, D.R., Hirsch, R.M. 2002, Statistical methods in water resources: U.S. Geological Survey Techniques of Water-Resources Investigations book 4, chap. A3, 522 p. https://doi.org/10.3133/twri04A3. Helsel, D.R., Hirsch, R.M., Ryberg, K.R., Archfield, S.A., and Gilroy, E.J. 2020. Statistical methods in water resources: U.S. Geological Survey Techniques and Methods, book 4, chap. A3, 458 p., https://doi.org/10.3133/tm4a3. [Super-sedes USGS Techniques of Water-Resources Investigations, book 4, chap. A3, version 1.1.] Herrington, C.S. 2017. Objective Zero: Improving the water quality mission objectives of the Watershed Protection Department. SR-18-02, City of Austin, Watershed Protection Department. https://services.austintexas.gov/watershed_protection/publications/document.cfm?id=292410 Jain V., Preston N., Fryirs K., Brierley G. 2006. Comparative assessment of three approaches for deriving stream power plots along long profiles in the upper Hunter River catchment, New South Wales, Australia. Geomorphology 74: 297–317. Johnson, B.E., Julien, P.Y., Molnar, D.K., Watson, C.C. 2000. The two-dimensional upland erosion model CASC2D-SED. Journal of the American Water Resources Association, 36: 31-42. https://doi.org/10.1111/j.1752-1688.2000.tb04246.x. Meyer-Peter, R., Müller, R. 1948. Formulas for bedload transport. Proceedings of the 2nd Meeting of the International Association of Hydraulic Research: Stockholm, 39–64. Knighton A.D. 1999. Downstream variation in stream power. Geomorphology 29: 293–306. Olinde, L., Lane, S., Richter, A., González, A., Scoggins, M. 2021, Stream corridors in the Blackland Prairie, City of Austin Watershed Protection Department, RR-21-01. City of Austin Watershed Protection Department. Orr, H.G., Large, A.R.G., Newson, M.D., Walsh, C.L. 2008. A predictive typology for characterizing hydromorphology. Geomorphology 100:32–40. Parker, G. 1990. Surface-based bedload transport relation for gravel rivers. Journal of Hydraulic Research 28: 417–436. https://doi.org/10.1080/00221689009499058. Phillips, R.T.J., Desloges, J.R. 2014. Glacially conditioned specific stream powers in low-relief river catchments of the southern Laurentian Great Lakes. Geomorphology 206: 271–287. https://doi.org/10.1016/j.geomorph.2013.09.030. Pike A.S., Scatena F.N., Wohl E.E. 2010. Lithological and fluvial controls on the geomorphology of tropical montane stream channels in Puerto Rico. Earth Surface Processes and Landforms 35(12): 1402–1417. RC&A. 1997. Technical Procedures Manual for Watershed Erosion Assessments: Final Report. Raymond Chan & Associates, Inc., 171p. https://services.austintexas.gov/watershed_protection/publications/document.cfm?id=186156. Reinfelds, I., Cohen, T., Batten, P., Brierley, G.. 2003. Assessment of downstream trends in channel gradient, total and specific stream power: a GIS approach. Geomorphology 60: 403–416. https://doi.org/10.1016/j.geomorph.2003.10.003. Sith, R., Nadaoka, K. 2017. Comparison of SWAT and GSSHA for high time resolution prediction of stream flow and sediment concentration in a small agricultural watershed. Hydrology, 4, 27. https://doi.org/10.3390/hydrology4020027. Van, L.N., Le, X.-H., Nguyen, G.V., Yeon, M., Jung, S., Lee, G. 2021. Investigating behavior of six methods for sediment transport capacity estimation of spatial-temporal soil erosion. Water 2021, 13, 3054. https://doi.org/10.3390/w13213054. Vaughan, I.P., Diamond, M., Gurnell, A.M., Hall, K.A., Jenkins, A., Milner, N.J., Naylor, L.A., Sear, D.A., Woodward, G., Ormerod, S.J. 2009. Integrating ecology with hydromorphology: a priority for river science and management. Aquat Conserv Mar Freshw Ecosyst 19:113–25. Zhang, H., Cao, Q., Ma, Y. 2023. Comparison of two hydro-sediment models based on energy principle of water movement in saturated/unsaturated soils. Energy Reports 9, 1561-1570. https://doi.org/10.1016/j.egyr.2022.11.138. Appendix A Change Log