20240807-004: WPD EII Quality Assurance Project Plan Part 3 — original pdf
Backup
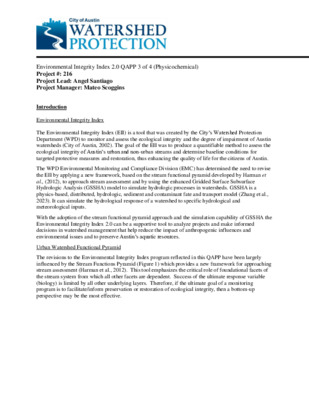
Environmental Integrity Index 2.0 QAPP 3 of 4 (Physicochemical) Project #: 216 Project Lead: Angel Santiago Project Manager: Mateo Scoggins Introduction Environmental Integrity Index The Environmental Integrity Index (EII) is a tool that was created by the City’s Watershed Protection Department (WPD) to monitor and assess the ecological integrity and the degree of impairment of Austin watersheds (City of Austin, 2002). The goal of the EII was to produce a quantifiable method to assess the ecological integrity of Austin’s urban and non-urban streams and determine baseline conditions for targeted protective measures and restoration, thus enhancing the quality of life for the citizens of Austin. The WPD Environmental Monitoring and Compliance Division (EMC) has determined the need to revise the EII by applying a new framework, based on the stream functional pyramid developed by Harman et al., (2012), to approach stream assessment and by using the enhanced Gridded Surface Subsurface Hydrologic Analysis (GSSHA) model to simulate hydrologic processes in watersheds. GSSHA is a physics-based, distributed, hydrologic, sediment and contaminant fate and transport model (Zhang et al., 2023). It can simulate the hydrological response of a watershed to specific hydrological and meteorological inputs. With the adoption of the stream functional pyramid approach and the simulation capability of GSSHA the Environmental Integrity Index 2.0 can be a supportive tool to analyze projects and make informed decisions in watershed management that help reduce the impact of anthropogenic influences and environmental issues and to preserve Austin’s aquatic resources. Urban Watershed Functional Pyramid The revisions to the Environmental Integrity Index program reflected in this QAPP have been largely influenced by the Stream Functions Pyramid (Figure 1) which provides a new framework for approaching stream assessment (Harman et al., 2012). This tool emphasizes the critical role of foundational facets of the stream system from which all other facets are dependent. Success of the ultimate response variable (biology) is limited by all other underlying layers. Therefore, if the ultimate goal of a monitoring program is to facilitate/inform preservation or restoration of ecological integrity, then a bottom-up perspective may be the most effective. Figure 1. Stream Functions Pyramid (Harman et al. 2012). A framework for approaching stream assessment. The Applied Watershed Research Section modified the stream functional pyramid, presented by Harman et al., (2012) (Figure 1), to adapt it more directly to the urban environment and watersheds as a unit instead of streams (Figure 2). The main difference is that Hydraulics and Geomorphology have been integrated into one layer. The urban stream functional pyramid includes four functional categories with the underlying controlling variables of geology and climate. Even though transitions between categories of the stream functional pyramid are not hard boundaries and cause-and-effect relationships can flow in both directions, the primary direction of cause-and-effect relationships flows from the bottom of the pyramid to the top, with functions higher on the pyramid more dependent on lower-level functions. Streams carry the water supplied by their watershed and groundwater. The resulting hydrology and hydraulic processes provide the foundation for all other functions that streams provide. The relationships between precipitation, runoff, infiltration, and groundwater flow determine the amount of water that the stream carries at any given time, the energy of the water to move sediment, the physicochemical processes that affect water quality, and the biological processes that the stream can support (Harman et al., 2012). Figure 2. EII 2.0 modified watershed functional pyramid. Each layer in this pyramid has a distinct modeling and monitoring approach. This implicitly includes geology as the underlying and fundamental framework for this pyramid, and the climate around it which drives the hydrologic cycle and many other factors that affect all layers. Physicochemical functions The physical and chemical characteristics of a stream are determining factors of aquatic ecosystem function and health. Physicochemical functions include physical and chemical processes that drive the basic water chemistry of the stream, regulating temperature and oxygen, breaking down the organic matter and carrying out the transformation of nutrients (Harman et al., 2012). These functions are highly influenced by each of the processes below it in the stream functional pyramid. Chemicals and fertilizers can move into a channel as water flows across a watershed, bank erosion caused by hydraulic forces can affect water clarity, oxygenation of water is affected by geomorphic bed diversity, and fast riffles, deep pools, and a dense riparian buffer are key to regulating water temperatures (Harman et al., 2012). Furthermore, moving upward in the stream functional pyramid, physicochemical functions highly influence biological functions. The functioning of an aquatic ecosystem and its stability to support life forms depend to a great extent on the physicochemical characteristics of its water (Kesete, 2021). Many aquatic organisms can be affected by small changes in habitat that can be caused by changes in water chemistry. Habitat incorporates all aspects of physical and chemical contaminants along with biotic interactions (Barbour et al., 1999b). The presence of an altered habitat structure is considered one of the major stressors of aquatic systems (Karr et al., 1986). Thus, physical and chemical parameters are pertinent to assess stream functions and aquatic habitat. Physicochemical water quality assessment parameters include nutrients (e.g., nitrogen and phosphorus), organic carbon, dissolved oxygen, temperature, pH, specific conductivity, and turbidity (Barbour et al., 1999a; Bernhardt et al., 2012; Gregory et al., 2019; Kaushal et al., 2015; Mahler et al., 2005, 2006; Moran et al., 2017; Van Metre et al., 2019). In between rain events, periods of little rain, or drought, water is introduced into the stream channel by influxes from delayed water pathways and groundwater exfiltration. The flow of water in the stream channel driven by these influxes is called baseflow. The baseflow plays an important role in hydrological processes and maintaining basic ecological functions of rivers and lakes and providing a source of water (Zhao et al. 2022). During baseflow periods, impurities are introduced into the aquatic system by different processes, such as groundwater exchange, weathering of rocks, leaching of soils and point and non-point sources of discharge (Patil, 2012). Knowledge about the baseflow can improve the understanding of catchment streamflow partitioning, assessing of surface-groundwater flow exchanges, conducting non- point-source pollution assessments, and aiding of water resource management (Zhao et al. 2022). In periods of rain, impurities are also introduced into the aquatic system by dissolution of aerosol particles from the atmosphere and from stormwater runoff (Patil, 2012). Stormwater runoff from the developed environment is one of the great challenges of modern water pollution control, as this source of contamination is a principal contributor to water quality impairment of waterbodies nationwide (National Research Council, 2009). In urban areas, the change in land use and the increase in impervious surface affect the hydrologic cycle by changing the relative percentage of precipitation that contributes to groundwater recharge, evapotranspiration, and runoff, causing stormwater runoff to increase in both rate and volume and to wash away more mass of pollutants that are introduced into the urban environment by vehicular traffic, industrial processes, building construction, and commercial and domestic activities (Gorgoglione et al., 2016). Chemical pollutants flushed out by surface runoff during storm events influence the physicochemical characteristics of the receiving water and can affect the aquatic habitats and stream function (Qin et al., 2016). Thus, an effective stormwater sampling program that generates data on chemical concentration and contaminant load entering streams system during storm events is critical to manage the functions they support. This data can be integrated with the GSSHA model, which also takes into account the influence of catchment characteristics such as surface area, land use and impervious surface, to simulate a watershed response to stormwater inputs. These simulation responses can be used in the EII 2.0 to help predict water quality conditions to facilitate stream preservation at watershed scale. Gridded Surface Subsurface Hydrologic Analysis (GSSHA) model Gridded Surface Subsurface Hydrologic Analysis (GSSHA) is a two-dimensional finite difference rainfall/runoff model. A finite difference grid is used to establish the computational domain and parameters for surface runoff. The GSSHA model is fully coupled with hydraulic stream flow/routing models (Downer, 2009). Parameters for stream channels are defined using arcs and then mapped to the appropriate underlying grid cells. GSSHA can be used as an episodic or continuous model where soil surface moisture, groundwater levels, stream interactions, and contaminant fate are continuously simulated (Zhang et al., 2023). The fully coupled groundwater to surface-water interaction allows GSSHA to model basins in both arid and humid environments. Basic simulated physical processes with GSSHA includes distributed rainfall, rainfall interception by vegetation, surface ponding and retention, infiltration, evapotranspiration, overland flow, streamflow, and lateral saturated groundwater flow (Downer, 2009). The model also simulates sub-surface drainage networks and includes seasonal effects related to snowfall/snowmelt and seasonal variability in plant transpiration and interception parameters. The model is intended for, and has been applied to the simulation of streamflow, flooding, soil moisture, sediment erosion and discharge. The hydrologic/hydraulic components of GSSHA provide the information necessary to simulate reactive contaminant transport. Key to simulating contaminants within a watershed, GSSHA provides the ability to link two-dimensional (2-D) overland flow transport to one-dimensional (1-D) transport in a stream network, though it is possible to simulate contaminants in the channels alone, if a point source of contaminants is introduced to the channel (Downer, 2009). Simulation of contaminants in the soil column requires that contaminants are simulated on the overland flow plane as well. Two types of reactive contaminant transport and fate are available in GSSHA: 1. Contaminants can be simulated as simple first order reactants with specified uptake rates from the 2. The nutrient cycle can also be simulated with the Nutrient Simulation Model (NSM) (Johnson soil and specified decay rates. and Gerald, 2008). The overall simulation methods for both types within the GSSHA model are the same, though the use of the first order contaminants requires explicit knowledge about the contaminants being simulated, and appropriate reaction rates, as all the reaction rates must be supplied to the model. Therefore, it is necessary to determine which method is best for the specific use. If nutrients are the contaminants of concern, then the NSM should be considered. If other contaminants need to be simulated, then they need to be simulated as first order reactants. It should be noted that static kinetic rates, or even first order rates, may not be appropriate for the reactants being considered. In addition, while the simple contaminants give great latitude in specifying and adjusting rates to match observed data, it is important to assure that the rates are reasonable for both the contaminants and conditions being simulated. While conceptually simple, the application of the simple contaminants actually requires more knowledge about the contaminants (i.e. chemistry) than the use of NSM, where the expert knowledge of the contaminants (phosphorous and nitrogen) has been programmed into the method. Project History The EII is a comprehensive biological, chemical, and physical monitoring tool designed to assess the ecological integrity and the comparative degree of impairment of Austin’s watersheds. It was originally developed and tested in the urban watersheds in 1994 and 1995 and initiated citywide in 1996. The methods of the original program were documented in Environmental Integrity Index Methodology (COA 1999). By the year 2000, water quality sampling was conducted quarterly, and the biological and habitat surveys were completed annually. Fifty of the City of Austin planning watersheds were grouped into three phases with approximately 150 total sites sampled on a three-year rotating basis (~50 sites sampled per year). Phase 1 primarily included the urban watersheds sampled historically under the Water Watchdog volunteer program (Project 20) while Phase 2 and Phase 3 included suburban and developing watersheds. In 2009, the watersheds were regrouped into two phases for sampling on a two-year rotating schedule. This regrouping was designed to increase site visit frequency to improve the resolution of temporal trend evaluation and align with frequency requirements of the Clean Rivers Program of the Texas Commission on Environmental Quality. Although the methods have remained relatively stable over time, the project has evolved slowly due to advancements in technology, staff bandwidth, and state-of-the-science. A retrospective of the project after 25+ years of work indicated that much could be gained with strategic advancements to the program. The EII has previously been used in the Department’s Master Plan process to evaluate the current water quality conditions of Austin’s watershed. Additionally, it has been used to satisfy a portion of the City’s National Pollution Discharge Elimination System (NPDES) permit. Perhaps the most valuable product of the EII is a long-term dataset that substantiates a baseline condition from which models can be developed. Objective Zero, a concept which WPD uses to identify target conditions for aspirational environmental goals (Herrington, 2017), could not be efficiently informed by the structure of EII as it existed in 2022. The 2022 fiscal year was the last year of traditional phase-based quarterly water quality sampling. Field and analysis methods were developed through four subcommittees for the EII 2.0: Hydrology, Geomorphology, Physicochemical and Biology. Each subcommittee evaluated the EII program for the past 30 years, reviewed the relevant body of work (both COA projects and literature), and discussed goals and aspirations to meet the needs (both current and future) of the rapidly growing community in the context of climate change and evolving environmental stressors. Previous Annual EII Reports • Water Watchdog Water Quality Report SR-94-01 • Environmental Integrity Index (EII) Phase 2 (2001) Watersheds Report SR-03-11 • Environmental Integrity Index Phase 1 (2003) Watersheds Report SR-06-04 • EII Phase II (2004) Watersheds Report SR-06-01 • Environmental Integrity Index Phase 3 (2005) Watershed Summary Report SR-06-10 • Environmental Integrity Index Phase 1 (2006) Watershed Summary Report SR-07-1 • Environmental Integrity Index Phase II Watershed Summary Report SR-08-10Environmental Integrity Index Phase 3 (2008) Watershed Summary Report SR-09-06 • Environmental Integrity Index Phase I and II (2009-2010) Watershed Summary Report SR-12-01 • Environmental Integrity Index Annual Report 2011-2012 SR-13-18 • Environmental Integrity Index Phase I and II (2013-2014) Watershed Summary Report SR-15-08 • Environmental Integrity Index Phase I & II (2015-2016) Watershed Summary Report SR-19-08 Previous EII Methodology and Related Reports • Environmental Integrity Index Methodology CM-99-01 • A review of surface water quality trends in streams and reservoirs in Austin, Texas: 1994-2018 SR-18-03 • Flow and impervious cover thresholds in aquatic communities in Austin SR-15-12 • Growth Trends and Environmental Integrity in Austin, Texas (2011) CN-11-02 • Creation of a multi-metric index for describing the environmental integrity of Austin-area lakes • Water Resource Evaluation Standard Operating Procedures Manual SR-04-04, revised 2015 (2011) SR-11-19 Project Objective The objectives of this QAPP are: stormflow conditions. 1. Collect water samples from spatially representative sites of Austin watersheds for baseflow and 2. Monitor and record continuous ambient water quality data (e.g., dissolved oxygen, water temperature, specific conductivity, and turbidity) during baseflow and stormflow conditions. 3. Calibrate and validate watershed models to create annual nutrient pollutographs using Contaminant Transport and Fate module of GSSHA (Gridded Surface Subsurface Hydrologic Analysis) model. Policy Relation From the 1970s, the City of Austin’s flood, erosion, and water quality protection efforts have matured with the development of comprehensive programs and management measures. The mission of the Watershed Protection for water quality protection is to protect and improve Austin’s waterways and aquifers for citizen use and the support of aquatic life. To assess water quality, WPD developed its first Environmental Integrity Index (EII) monitoring and scoring system. The EII has been used by the City as an overall indicator of watershed ecological integrity and as a reference to evaluate and prioritize comprehensive water quality programs and feasible solutions to address water quality problems in Austin. But with population and development increasing at a fast pace in Austin, and the increasing sophistication of WPD watershed science, a revision of the EII was necessary. Results from the EII 2.0 monitoring program and physicochemical data (modeled data, calibration data and verification data) can be used to: • Support the department’s Strategic Plan (Rain 2 River). • Support the department’s Watershed Health Decision Support System (WHDSS), which is focused on problem identification, prioritization, and solution planning. • Provide targeted water quality data (physicochemical modeled data and scores) to a wide range of internal and external stakeholders at a very high resolution in all of Austin’s jurisdictional watersheds. Project Committee The EII 2.0 project has four separate QAPP committees structured in accordance with the modified functional pyramid: Hydrology, Hydraulics/ Geomorphology, Physicochemical, and Biology. The physiochemical committee structure (ARCI) is detailed in Table 1. Table 1. EII 2.0 Physiochemical QAPP Committee Structure Role Personnel Planning Data Analysis Modeling Mateo Scoggins Angel Santiago Robert Clayton Project Manager Project Lead Data Manager Committee Member (DA/DS) Abel Porras Committee Member (DA/DS) Young-Hoon Jin Ryan Burke Committee Member (AWR) Zhen Xu Committee Member (AWR) Christina Bryant Committee Member (AWR) Andrew Clamann Committee Member (AWR) Julia Siegmund Committee Member (AWR) Scott Hiers Committee Member (ASC) Brent Bellinger Committee Member (AWR) Radmon Rice Committee Member (ASC) Committee Member (AWR) Bianca Perez Committee Member (DA/DS) Yazmin Avila Committee Member (DA/DS) Andrew Chu Collection A R R C I R C C C C I I R C C I A R I R C I C I C I I I I I I I A C I R R I I I C I I I I I C C * A = Accountable (ensures project has needed resources, held accountable if not completed); R = Responsible (leads development of study components, performs and delegates tasks, reviews deliverables before deemed complete); C = Consulted (specialist in specific component of study, available for comments); I = Informed (kept in the loop, comments optional) Personnel Time A R C R C R R C C C C C C C C C Report Writing A R I R R C C C C I I I C I C I Table 2. Estimated Time Budget Task Project Management Site Design and Construction* Pre-Storm Prep Storm Event Monitoring Post-Storm Collection Field Equipment Maintenance* Data Management Modeling Reporting Personnel Angel Santiago AWR AWR AWR AWR AWR AWR, DA/DS AWR, DA/DS AWR Work Time Estimated 80hrs annually Estimated 500hrs annually Estimated 70hrs annually Estimated 25hrs annually Estimated 125hrs annually Estimated 500hrs annually Estimated 250hrs annually Estimated 500hrs annually Estimated 40hrs annually * Parts of data collection and equipment used for this QAPP align with those in the EII 2.0 Hydraulic- Geomorphology QAPP and EII 2.0 Hydrology QAPP. Project Funding Table 3. Project Funding Sources FDU (Fund Department Unit) 5100 6300 3135 (Lab, Analysis) 5100 6300 3140 (Field) Project Schedule Table 4. Project Schedule Target Discrete stormflow sample collection Composite stormflow sample collection Baseflow sample collection Field data QA/QC Modeling and data analysis Annual report drafting Annual report review Parameters FY24- Q1 FY24- Q2 FY24- Q3 FY24- Q4 FY25-Q1 Oct-24 FY25-Q1 Nov-24 2 storm events (one ≥ 1″ rain and the other < 1″ rain) FY25- Q1 Dec- 24 ✓ ✓ ✓ ✓ ✓ ✓ ✓ ✓ ✓ ✓ ✓ ✓ ✓ ✓ ✓ • Nutrients o Nitrogen: ▪ Samples to DHL: • NO3NO2-N by EPA method 300. • TKN by EPA method 351.2. • Ammonia-N by Standard Methods 4500-NH3-D. ✓ ✓ ✓ ▪ Samples to the USGS lab: an alkaline persulfate digestion approach for total nitrogen (report.pdf (usgs.gov)). o Total Phosphorus by Standard Methods 4500-PE. o Use acid-preserved containers. o Holding time of 28 days with acid-preservation and stored at 2-4 °C. o Samples to DHL Analytical will be delivered in ISCO containers after collection. o Samples to USGS will be collected and delivered by USGS staff. • In-situ multiparameter data sonde measurements will be collected at 15 minute interval for continuous monitoring of dissolved oxygen, temperature, specific conductivity, and turbidity in accordance with the WPD Environmental Monitoring & Compliance Standard Operating Procedures Manual 2023. • E. coli by Standard Methods 9223 (only for baseflow sampling). Field Methods Sampling Locations Candidate monitoring sites were selected by hierarchical cluster analysis (Table 5) (Figure 3), which seeks to partition all identified nodes in Austin watersheds into distinct groups with similar characteristics. The cluster analysis used the following watershed characteristics: • Infiltration total volume, mean volume and standard deviation of infiltration volume based on Soil Survey Geographic Database (SSURGO) soil infiltration data. • Annual total nitrogen and phosphorus loading. • Areal slope, buffer stream slope, and stream slope. • Percent impervious cover. • Watershed (catchment) size. Eight storm sites (Table 5) were identified using hierarchical clustering. One additional site, Gilleland @ FM 973 (GIL), was added to analyze the effects of wastewater discharges from treatment facilities to the receiving water. Field evaluations at the sites were performed to determine suitability for access and installation of monitoring station. The data obtained from stormflow and baseflow monitoring at these 9 sites will be used to calibrate the stormflow and baseflow Contaminant Transport and Fate module of the GSSHA model. The preference is to input the new EII 2.0 sites to existing sites in the WRM database, so new data can be compared to historic data. All EII 2.0 sites will be input as the nearest existing EII 1.0 site unless a site does not exist at that location or exceeds a reasonable distance (~50 feet) to be listed as the same site. If this occurs, a new site will be added to the database. Note that since site equipment must be mounted near solid structures, in some instances this tolerance was exceeded. One shelter will be constructed at each monitoring site for equipment, the locations of which are constrained by hydrological conditions of sites (no floodwater to batteries and wiring), maximum tubing length of the autosampler pump (100 feet), and maximum head of the autosampler pump (28 feet). Baseflow sampling will be also performed at selected long-term sites and randomly selected sites (Table 5) to validate the baseflow Contaminant Transport and Fate module of the GSSHA model. Long-term sites are those that have an extensive history of monitoring within the EII program and have stable suitable riffle habitat. For randomly selected sites, at the beginning of each fiscal year, a list of 10 random sites from each of the eight modelled clusters plus one wastewater-driven stream (total of 90 sites) shall be generated resulting in a stratified random list. At least one site for each of the eight modelled clusters in addition to one site characterizing wastewater-driven streams will be selected. Reconnaissance of random sites will be conducted to assure that at least one site for each cluster is physically accessible (this may require contacting property owner) and safe. If sites are dry or otherwise unsampleable, additional random sites from the list may be sampled. Table 5. Sample site list Stormwater Long term Random** Cluster Site ID Site Short Name Barton @ Lost Creek Blvd Bull @ Spicewood Springs Rd 7th Crossing Elm @ FM969 Gilleland @ FM 973 Onion @ Ped Xing south of William Cannon Rinard @ Bradshaw Rd Slaughter @ FM1826 51 142 3068 1192 2956 233 623 5285 Walnut @ Metric Blvd 463 Wells Branch @ Walnut Creek Park Rd Blunn @ Riverside 180 Boggy @ Manor 2754 Marble @ William Cannon 231 Onion @ FM 150 612 1366 Onion @ SAR 624 Waller @ 23rd TBD TBD TBD TBD TBD TBD TBD TBD TBD Annual random site representing Cluster 1 Annual random site representing Cluster 2 Annual random site representing Cluster 3 Annual random site representing Cluster 4 Annual random site representing Cluster 5 Annual random site representing Cluster 6 Annual random site representing Cluster 7 Annual random site representing Cluster 8 Annual random site representing ww effluent X X X X X X X X X X X X X X X X X X ID 2 4 5 5 * 8 1 3 6 7 7 7 1 * 8 7 1 2 3 4 5 6 7 8 TBD* X X X X X X X X X * Although site 1192 and a random site are located within one of the eight clusters of the model, these sites have been selected to characterize stream segments that are wastewater effluent driven with unique hydrology and chemical characteristics. ** Random sites shall be selected at the beginning of each fiscal year, site coordinates to be added to Appendix A. Figure 3. Sample site location map of the nine stormwater stations and nine routine long-term stations (three of which overlap with stormwater). The nine randomly selected sample sites are not shown. Data Collection ISCO Signature Flow Meter: Designed for continuous open channel stage measurements. At each monitoring site, the Flow Meter will measure stage utilizing one or more of the following methods: bubbler level, ultrasonic elevation, or laser level with velocity readings. The stage measured by the flow meter will be used to signify rainfall-runoff events and will trigger the autosamplers to enable and begin collecting stormwater chemistry samples. A BLZZRD™ portable refrigerated autosampler and ISCO 3700 autosampler will be installed at each monitoring site for water sample collection. The ISCO Signature® flow meter triggers the autosamplers initiate collection and controls pacing. When a stage threshold is reached, the autosamplers will enable and collect the sample per the programed sample pacing of 15 minutes. A maximum of 14 discrete samples per event will be collected by the sampler and delivered to the analytical lab after each event. Aqua TROLL 500 data sondes will be deployed at each monitoring station for in-situ water quality measurements. The Aqua TROLL 500 is a fully customizable multiparameter sonde with the capacity for four interchangeable sensors. Sensors will include temperature, specific conductivity, rugged dissolved oxygen (RDO®), and turbidity with a wiper also present. The Aqua TROLL will be directly connected to the ISCO Signature Flow Meter via a vented twist-lock cable where the meter will upload the sonde data to Flowlink along with the hydrology and sample collection data on a set daily schedule. Data collected from in-situ monitoring and sample analysis during the initial years will be primarily used for model calibration. The QAPP committee will regularly assess progress. Once the model calibration aligns with the committee's standards, the QAPP will undergo revisions to incorporate new elements into sampling locations, sampling schedule, laboratory costs and analytical methods sections for a validation phase. Sampling schedule • Stormflow sampling o The annual goal is to collect nutrient samples in 6 storm events at each of the 9 monitoring sites (Table 9) for the calibration needs of the Contaminant Transport and Fate module of GSSHA model. ▪ Two Storm events will be discreet sampling. Each discreet sampling event will collect up to 14 discrete nutrient samples per BLZZRD™ portable refrigerated autosampler. • For 1 event, samples will be collected with precipitation greater than 1 inch. • For 1 event, samples will be collected with precipitation less than 1 inch. ▪ Four storm events will be composite sampling. Each composite sampling will be collected following TCEQ seasonal monitoring periods. Each monitoring site will collect up to 14 aliquots that will be composited into one nutrient sample. o Season 1 (Wet): September – October o Season 2 (Dry): November – February o Season 3 (Wet): March – June o Season 4 (Dry): July – August ▪ All sites will not necessarily have samples collected during the same event. If a season is missed, it will be resampled the following year. o AWR automated stormwater samples will be time weighted. • 15-minute intervals. • Total of 3.5 hours. o USGS automated sampling will be flow weighted. • Baseflow sampling ▪ The annual goal is to collect 1 baseflow grab sample at all monitoring sites on a quarterly basis for nutrient and E. coli analysis (Total of 4 samples per site per year). ▪ Baseflow sampling will be performed according to the Environmental Monitoring and Compliance Standard Operating Procedures Manual of 2019. Laboratory Analyses Table 6. Laboratory cost Lab Annual or Total Cost DHL Analytical USGS Lab $46,400 $107,600 For the current contract with DHL: • • • • • item 1.1 (nitrite and nitrate nitrogen by EPA method 300), $20 per sample. Item 1.2 (ammonia nitrogen by Standard Methods 4500-NH3-D), $24 per sample. item 1.4 (total phosphate by Standard Methods 4500-PE), $29 per sample. item 1.5 (TKN by EPA method 351.2, subcontracted to another lab), $57 per sample. Item 1.10 (E. coli by Standard Methods 9223), $50 per sample. For number of samples to be collected in FY 24: • 9 monitoring sites (2 handled by USGS for stormflow sampling). • 2 discrete stormflow sampling events with 14 samples per event. • 4 composite stormflow sampling events with 1 sample per event. • 4 baseflow sampling events with 1 grab sample per event. Total FY 24 budget estimate: • For DHL Analytical: o Stormflow sampling: 7 × (14 × 2 + 4) × ($20 + $29 + $57 + $24) = $29,120 o Baseflow sampling: 24 × 4 × ($20 + $29 + $57 + $24 + $50) = $17,280 • For the contract with USGS: o Site 8155240 Barton Ck at Lost Ck Blvd nr Austin, TX: $53,800 o Site 08158840 Slaughter Ck at FM 1826 nr Austin, TX: $53,800 o Total: $107,600 QC Specification Quality Assurance (QA) and Quality Control (QC) methods are critical components of water quality sampling schemes. These methods ensure data collected is accurate, reliable, and of high quality. The following are some common QA/QC methods used in water quality sampling: 1. Calibration: Instruments used for field measurements should be calibrated to ensure accuracy. a. Calibration of the sensors will be according to SOP guidelines which are reflective of, and well within, TCEQ SWQM requirements. i. If a sensor is discovered to have drifted outside of the acceptable SOP sensor range, the values will be adjusted on a sloped scale from the last calibration. A caveat here is if there is a point in time that an operator can identify that would have caused the drift, then the adjustment will be made from that point according to the best judgement by the operator. Please see USGS paper on adjusting time- series data. 2. Sample collection and preservation will be completed in accordance with WPD Standard Operating Procedures (SOPs): Stormwater Monitoring SOP a. Pre-Storm Event: When a qualifying rain event is anticipated, DHL/USGS pre clean 950mL bottles will be placed in the BLZZRD and ISCO 3700 autosamplers at each site. 2mL of H2SO4 will be added to each bottle of the BLZZRD autosampler for sample preservation. b. Post-Storm Event: After a qualifying rain event and when conditions are safe to do so, the bottles will be collected from the autosamplers at each site within one week from the last aliquot collection time, not to exceed holding times of 28 days. Bottles will be capped with clean lids and placed on ice and cooled to <2 °C for transport to DHL Monday- Thursday between 0800 and 1400 (for COA managed sites) or the USGS Kentucky Sediment Laboratory for analysis (for USGS managed sites). c. Sample volume of the autosamplers will be checked and adjusted on a quarterly basis. 3. Chain of Custody: A chain of custody (COC) is the record of ownership for water quality samples to ensure sample integrity. Personnel collecting samples from autosamplers for laboratory analysis will generate a COC utilizing the Water Resources Monitoring (WRM) database prior to sample collection. Each monitoring site and sampling event will have a unique COC with the following information: a. Site number, name, and description. b. If the samples are to be composited or analyzed as discrete. c. Sample ID number. d. Sample date. e. sample time. The COC will be printed and delivered to the lab along with the water quality samples. Laboratory personnel and COA scientists will sign and date the COC documenting the relinquish and acceptance of sample custody. See the WRM User Guide for more information. Validation: Data validation is the process of checking the data for errors or inconsistencies. Data validation includes checking for outliers and ensuring that data meets quality control criteria. See the Stormwater Monitoring Workflow for more information. Analytical Methods E. coli predictive modeling WPD has been collecting E. coli measurements for over 15 years. This data was mathematically summarized at each EII 1.0 site using regulatory methods (geomeans and single sample measurements) and then partitioned into three categories: Acceptable, Episodic, and Chronic. Acceptable sites were deemed to comply with regulatory requirements. Episodic sites were assumed to be out of compliance, but only due to a small number of high measurements. Chronic sites had consistently high measurements of E. coli concentrations (Porras and Kocian, 2020). Watershed site characteristics at each EII 1.0 site and their respective partitioned target categories were used to train various supervised machine learning algorithms. Testing these algorithms resulted in an accuracy of about 66%. Given this fair output, the algorithms were used to predict E. coli categories for each of the 3000 EII 2.0 sites. Future field observations of E. coli at EII 2.0 sites will be used to validate the algorithms. Reactive Contaminant Transport Reactive contaminant transport can be simulated on the overland flow plane, in channels and reservoirs, and in the soil. Contaminants move in 2-Dimensions in the overland flow, with reactions occurring as water moves across the watershed, and in one dimension (1-D) in the channel network using the reactive advection dispersion equation. • Specifically for 1-D channel transport, contaminants will be simulated as simple first order reactants with specified mass transfer rates from the soil and specified decay rates. The decay coefficient can be set as a uniform value for every stream node by specifying the value (Time-1). In addition to setting the rate, users can also define the dispersion coefficient (Length2 Time-1) and initial concentrations (Mass Length-3). The general 1-D advection-dispersion equation is used for reactive transport in channels: 𝜕(𝑀) 𝜕𝑡 ) + 𝐾(𝑀) = 𝑆 (1) 𝜕(𝑢𝑀) 𝜕𝑥 𝜕𝑀 𝜕𝑥 𝜕 𝜕𝑥 (𝐷 + − where u is flow velocity in the x-direction (Mass Time-1), M is the mass of contaminant that is equal to the concentration (Mass Length-3) multiplied by the volume (Length3), K is the decay coefficient (Time-1), and S is the term for all sources and sinks (Mass Time-1). This equation is solved with a simple three-point explicit finite-volume scheme for each contaminant of concern. The solution initiates at the most upstream node and proceeds downstream to the channel outlet. This scheme is first order accurate in time and space. For each node, the following mass reactions are accounted for with masses (M) in grams; volumes (V) in m3; discharges (Q) in m3 s-1; and concentrations (C) in g m-3 or mg L-1 (Downer, 2009). For the channels, the following sources/sinks are considered in addition to chemical reactions: 1. Exchange with overland flow. 2. Exchange with reservoirs. 3. Exchange with groundwater. 4. Point sources. • The 2-D form of the general transport equation is used for overland flow: 𝜕(𝑀) 𝜕𝑡 + 𝜕(𝑢𝑀) 𝜕𝑥 + 𝜕(𝑣𝑀) 𝜕𝑦 − 𝜕 𝜕𝑥 (𝐷𝑥 𝜕𝑀 𝜕𝑥 ) − 𝜕 𝜕𝑦 (𝐷𝑦 𝜕𝑀 𝜕𝑦 ) + 𝐾(𝑀) = 𝑆 (2) where u is flow velocity in the x-direction (Mass Time-1), v is the velocity in the y-direction (Mass Time-1), M is the mass of contaminant that is equal to the concentration (Mass Length-3) multiplied by the volume (Length3), K is the decay coefficient (Time-1), and S is the term for all sources and sinks (Mass Time-1). For the 2-D overland flow plane, different from channel transport, uptake from land surface and soil can take place in addition to decay. Candidate input parameters include: • Dispersion coefficient on the overland (Length2 Time-1) • Soil and Overland Decay Coefficient (Time-1)) • Uptake coefficient Ku (Length Time-1) Initial loading (Mass) or (Mass Mass-1) • • Groundwater concentration (Mass Length-3) • • Soil water distribution coefficients in the soil Kd (Length3 Mass-1) • Solubility Cmax (Mass Length-3) Initial concentration (Mass Length-3) Assumptions For the purpose of this project, general modelling assumptions are as follows: • Contaminants are assumed to be within the overland flow plane and the soil column. For contaminants in the soil column, the mass of contaminants is distributed over a specified mixing depth in the soil column. • As rainfall accumulates on the overland flow plane, uptake of contaminants occurs as contaminants on the overland flow plane are dissolved and/or contaminants in the soil column diffuse into the overlying surface waters. • Some (or all) of the water ponded on the land surface may infiltrate, removing contaminants. Water that infiltrates is assumed to contain the same concentration of dissolved contaminants as the ponded water. • Contaminants are not simulated in the groundwater. Concentration of contaminants is specified for every cell in the groundwater domain. If the groundwater table is high enough, water may spill out onto the overland flow plane as exfiltration. The concentration of contaminant in the water spilling back on the overland flow plane will be calculated as part of the soil constituent transport. The water that enters the soil column from the bottom is assumed to have the specified groundwater concentrations for the contaminant in that cell. Contaminants seeping out of the soil column into the groundwater are accounted for but do not affect the static groundwater concentration for the cell. • The dispersion and decay rates are uniform throughout the grid. • In general, the overland flow plane acts as the primary source of contaminants to the channel, which is a sink for the overland flow plane and a source for the channel. For cases where overbank flooding occurs, the stream may overflow and add water, as well as contaminants, back to the overland flow plane. • Groundwater provides a source of contaminants to the channel if baseflow occurs. Concentration of contaminants is specified for every cell in the groundwater domain. This concentration does not vary throughout the simulation. If flow is from the groundwater domain to the channel, water entering the channel is assumed to have the specified groundwater concentrations of contaminants. Seepage from the channel to the groundwater is assumed to have the same concentration as the water in the channel node. Additions and subtractions to the groundwater are accounted for but do not affect the specified groundwater concentrations. • Default values for initial channel concentration (g m-3), channel decay (d-1), and channel dispersion coefficient (m2s-1) are zero but can be adjusted for one contaminant per simulation. • Particulate phosphorus accounts for total phosphorus. • Organic nitrogen = TKN – ammonia-N. Reporting An annual report will be prepared to summarize progress in data collection and whether model needs are met. A decision will be made then on whether sampling strategies need to be adjusted for the following sample year. Raw data will be reposited in the WRM database and made available through Socrata portal. Information will be available to the public via a web-based interactive map (www.atxwatersheds.com/findyourwatershed/ or similar) with the most current modelled results. Raw data will be reposited in the WRM database and made available through Socrata portal. Distribution The most recent QAPP is available in the WPD SharePoint. The project lead is responsible for informing all committee members via email if significant changes are made to the QAPP. Change Procedures The Project Lead will maintain this QAPP. Proper document management procedures are followed during any update and the QAPP will be locked for editing. The original approved QAPP will be kept as documentation and any changes to the QAPP are summarized in the QAPP Revision History below. Table 7. QAPP Acceptance Subcommittee Hydraulics/Geomorphology Project Lead (QAPP 2 of 4) Zhen Xu Role Project Manager Project Lead DA/DS Supervisor Data Manager Committee Member (AWR) Biology Project Lead (QAPP 4 of 4) Committee Member (ASC) Hydrology Project Lead (QAPP 1 of 4) Committee Member (DA/DS) Committee Member (DA/DS) Table 8. QAPP Revision History References Date Modified By Modification Description Mateo Scoggins 11/14/2023 (!) Name Angel Santiago Abel Porras Robert Clayton Ryan Burke Date of Acceptance 11/14/2023 11/14/2023 11/14/2023 11/14/2023 11/08/2023 11/14/2023 Andrew Clamann 11/13/2023 Rice Radmon Christina Bryant 11/13/2023 Yazmin Avila 11/14/2023 Young-Hoon Jin 11/14/2023 Date of QAPP Acceptance Subcommittee approval Barbour, M. T., Gerritsen, J., Snyder, B. D., & Stribling, J. B. (1999a). Rapid bioassessment protocols for use in streams and wadeable rivers. USEPA, Washington. Barbour, M. T., Gerritsen, J., Snyder, B. D., & Stribling, J. B. (1999b). Rapid bioassessment protocols for use in streams and wadeable rivers: periphyton, benthic macroinvertebrates and fish, Second Edition. In US Environmental Protection Agency Office of Water Washington DC (Vol. 2nd). https://doi.org/EPA 841-B-99-002 Bernhardt, E. S., Band, L. E., Walsh, C. J., Berke, P. E., Carey, R. O., Hochmuth, G. J., Martinez, C. J., Boyer, T. H., Dukes, M. D., Toor, G. S., Cisar, J. L., Fissore, C., Hobbie, S. E., King, J. Y., McFadden, J. P., Nelson, K. C., Baker, L. a. L. a., Houdeshel, C. D., Hultine, K. R., … Hunt, W. F. (2012). Review of Dissolved Pollutants in Urban Storm Water and Their Removal and Fate 6609–6624. Water https://doi.org/10.1061/(ASCE)EE.1943-7870.0000876. Bioretention Research, 135(1), Cells. in City of Environmental http://www.austintexas.gov/watershed_protection/publications/document.cfm?id=186267 Integrity (2002). Austin. Index Methodology. Downer, C. W. (2009). Simulation of Reactive Contaminant Fate and Transport in Hydrologic Simulator GSSHA. Gorgoglione, A., Gioia, A., Iacobellis, V., Piccinni, A. F., & Ranieri, E. (2016). A Rationale for Pollutograph Evaluation in Ungauged Areas, Using Daily Rainfall Patterns: Case Studies of the Apulian Region in Southern Italy. Applied and Environmental Soil Science, 2016. https://doi.org/10.1155/2016/9327614 Gregory, L. F., Gitter, A., Muela, S., & Wagner, K. L. (2019). Should Contact Recreation Water Quality Standards be Consistent across Hydrological Extremes? Journal of Contemporary Water Research & Education, 166(1), 12–23. https://doi.org/10.1111/j.1936-704x.2019.03298.x Harman, W., Starr, R., Carter, M., Tweedy, K., Clemmons, M., Suggs, K., & Miller, C. (2012). A Function- Based Framework for Stream Assessments and Restoration Projects (EPA 843-K-12-006; Issue May). Herrington, C.S. 2017. Objective Zero: Improving the water quality mission objectives of the Watershed Protection Department. SR-18-02, City of Austin, Watershed Protection Department. https://services.austintexas.gov/watershed_protection/publications/document.cfm?id=292410 Karr, J. R., Fausch, K., Angermeier, P. L., Yant, P. R., & Schlosser, I. J. (1986). Assessing biological rationale. method and its in integrity running https://www.researchgate.net/publication/245507787 waters. A Kaushal, S., McDowell, W., Wollheim, W., Johnson, T., Mayer, P., Belt, K., & Pennino, M. (2015). Urban evolution: the role of water. Water, 7(8), 4063–4087. https://doi.org/10.3390/w7084063 Kesete, N. (2021). Water Quality Assesment of Chole Stream Using Some Physico Chemical Parameters and Water Quality Index. Journal of Community Medicine & Health Education, 11(9). Mahler, B. J., Van Metre, P. C., Bashara, T. J., Wilson, J. T., & Johns, D. A. (2005). Parking lot sealcoat: An unrecognized source of urban polycyclic aromatic hydrocarbons. Environmental Science and Technology, 39(15), 5560–5566. https://doi.org/10.1021/es0501565 Mahler, B. J., Van Metre, P. C., Wilson, J. T., Guilfoyle, A. L., & Sunvison, M. W. (2006). Concentrations, Loads, and Yields of Particle-Associated Contaminants in Urban Creeks, Austin, Texas, 1999-2004. In Scientific Investigations Report 2006–5262. Moran, P. W., Nowell, L. H., Kemble, N. E., Mahler, B. J., Waite, I. R., & Van Metre, P. C. (2017). Influence of sediment chemistry and sediment toxicity on macroinvertebrate communities across 99 wadable streams of the Midwestern USA. Science of the Total Environment, 599– 600(December), 1469–1478. https://doi.org/10.1016/j.scitotenv.2017.05.035 National Research Council. (2009). Urban stormwater management in the United States. In Urban States. National Academies Press. the United in Stormwater Management https://doi.org/10.17226/12465 Patil, P. N. (2012). Physicochemical parameters for testing of water-A review. In INTERNATIONAL 3). ENVIRONMENTAL SCIENCES (Vol. Issue OF 3, JOURNAL https://www.researchgate.net/publication/344323634  Porras, A., Kocian, L. (2020). Towards a Mathematically-based Assessment of Bacteriological Water Quality in Austin’s streams. SR-20-01, City of Austin, Watershed Protection Department. https://services.austintexas.gov/watershed_protection/publications/document.cfm?id=346149 Qin, H. peng, He, K. mao, & Fu, G. (2016). Modeling middle and final flush effects of urban runoff Journal of Hydrology, 534, 638–647. an urbanizing in pollution catchment. https://doi.org/10.1016/j.jhydrol.2016.01.038 Van Metre, P. C., Waite, I. R., Qi, S., Mahler, B., Terando, A., Wieczorek, M., Meador, M., Bradley, P., Journey, C., Schmidt, T., & Carlisle, D. (2019). Projected urban growth in the southeastern USA 1–17. https://doi.org/10.1371/journal.pone.0222714 streams 14(10), ONE, PLoS small risk. puts at Zhang, H., Cao, Q., & Ma, Y. (2023). Comparison of two hydro-sediment models based on energy principle of water movement in saturated/unsaturated soils. Energy Reports, 9, 1561–1570. https://doi.org/10.1016/j.egyr.2022.11.138 Zhao G, Kong L, Li Y, Xu Y, Li Z. (2022). Investigating Historical Baseflow Characteristics and Variations in the Upper Yellow River Basin, China. Int J Environ Res Public Health, 19(15):9267. doi: 10.3390/ijerph19159267. PMID: 35954621; PMCID: PMC9367871.  Appendix A Change Log