20201021-002a: City of Austin Comments on Sawyer-Cleveland Wastewater Treatment Plant — original pdf
Backup
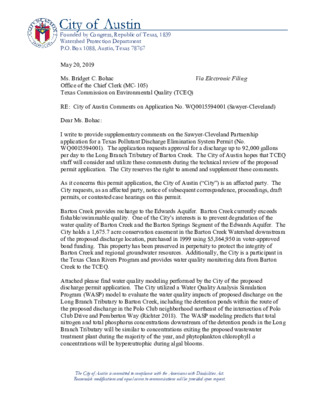
Via Electronic Filing Founded by Congress, Republic of Texas, 1839 Watershed Protection Department P.O. Box 1088, Austin, Texas 78767 May 20, 2019 Ms. Bridget C. Bohac Office of the Chief Clerk (MC-105) Texas Commission on Environmental Quality (TCEQ) RE: City of Austin Comments on Application No. WQ0015594001 (Sawyer-Cleveland) Dear Ms. Bohac: I write to provide supplementary comments on the Sawyer-Cleveland Partnership application for a Texas Pollutant Discharge Elimination System Permit (No. WQ0015594001). The application requests approval for a discharge up to 92,000 gallons per day to the Long Branch Tributary of Barton Creek. The City of Austin hopes that TCEQ staff will consider and utilize these comments during the technical review of the proposed permit application. The City reserves the right to amend and supplement these comments. As it concerns this permit application, the City of Austin (“City”) is an affected party. The City requests, as an affected party, notice of subsequent correspondence, proceedings, draft permits, or contested case hearings on this permit. Barton Creek provides recharge to the Edwards Aquifer. Barton Creek currently exceeds fishable/swimmable quality. One of the City’s interests is to prevent degradation of the water quality of Barton Creek and the Barton Springs Segment of the Edwards Aquifer. The City holds a 1,675.7 acre conservation easement in the Barton Creek Watershed downstream of the proposed discharge location, purchased in 1999 using $5,864,950 in voter-approved bond funding. This property has been preserved in perpetuity to protect the integrity of Barton Creek and regional groundwater resources. Additionally, the City is a participant in the Texas Clean Rivers Program and provides water quality monitoring data from Barton Creek to the TCEQ. Attached please find water quality modeling performed by the City of the proposed discharge permit application. The City utilized a Water Quality Analysis Simulation Program (WASP) model to evaluate the water quality impacts of proposed discharge on the Long Branch Tributary to Barton Creek, including the detention ponds within the route of the proposed discharge in the Polo Club neighborhood northeast of the intersection of Polo Club Drive and Pemberton Way (Richter 2018). The WASP modeling predicts that total nitrogen and total phosphorus concentrations downstream of the detention ponds in the Long Branch Tributary will be similar to concentrations exiting the proposed wastewater treatment plant during the majority of the year, and phytoplankton chlorophyll concentrations will be hypereutrophic during algal blooms. a The City of Austin is committed to compliance with the Americans with Disabilities Act. Reasonable modifications and equal access to communications will be provided upon request. chris.herrington@austintexas.gov or at Founded by Congress, Republic of Texas, 1839 Watershed Protection Department P.O. Box 1088, Austin, Texas 78767 The results from the WASP model were utilized in an analytic model to evaluate the potential water quality impacts of the proposed discharge on the mainstem of Barton Creek (Porras 2019). The analytic model predicts that, during low flow conditions, Barton Creek periphytic chlorophyll levels for a distance up to 15.6 miles downstream of the confluence of Barton Creek and the Long Branch Tributary. This change in trophic status would degrade water quality by more than a and would degrade the quality of water recharging the Edwards Aquifer. The City hopes that TCEQ staff will consider these comments during this process. Thank you for your consideration, and please contact me at 512-974-2840 if you have any questions. Sincerely, de minimus extent, would adversely impact existing recreational and aquatic life uses, a concentrations would be degraded from oligotrophic to mesotrophic Christopher S. Herrington, P.E., Environmental Officer City of Austin cc: Patricia Link, Assistant City Attorney, City of Austin The City of Austin is committed to compliance with the Americans with Disabilities Act. Reasonable modifications and equal access to communications will be provided upon request. Analysis of a Proposed Wastewater Treatment Plant Discharge to the Long Branch Tributary of Barton Creek DR-18-08; November 2018 Aaron Richter City of Austin Watershed Protection Department Environmental Resource Management Division ABSTRACT The Water Quality Analysis Simulation Program (WASP) is commonly used to model water quality responses to wasteloads. The City of Austin used the WASP model to create a continuous simulation of a proposed wastewater treatment plant (WWTP) discharge to the Long Branch Tributary of Barton Creek. The discharge would enter 5 detention ponds prior to entering the Long Branch Tributary. Results of the WASP model show that total nitrogen (TN) and total phosphorus (TP) concentrations downstream of the detention ponds will be similar to concentrations leaving the WWTP during the majority of the year and phytoplankton concentrations will be at hypereutrophic levels during blooms. Nutrients and chlorophyll a concentrations should be used as input into a parsimonious model to determine how far downstream the impacts will travel. INTRODUCTION The Sawyer-Cleveland Partnership applied to the Texas Commission on Environmental Quality (TCEQ) for a new Texas Pollutant Discharge Elimination System (TPDES) permit to discharge treated wastewater effluent into the Barton Creek Watershed in the Contributing Zone of the Barton Springs Segment of the Edwards Aquifer (Proposed Permit No. WQ0015594001, EPA I.D. No. TX0137863). The proposed permit would authorize a discharge of treated wastewater not to exceed a daily average flow of 0.092 MGD (92,000 gallons per day). The location of the proposed wastewater treatment plant (WWTP) is approximately 220 m (720 ft) southwest of the intersection of US Highway 290 and Sawyer Ranch Rd. (Figure 1). The proposed discharge would travel through a series of detention ponds, approximately 9.771 km (32,057 ft; 6.1 miles) through the Long Branch Tributary to Barton Creek, and approximately 37.945 km (124,589 ft; 23.6 miles) through Barton Creek to the boundary of the Recharge Zone. The permit application requested treatment standards of 10 mg/L 5-day Biochemical Oxygen Demand (BOD5), 15 mg/L total suspended solids (TSS), 2 mg/L ammonia nitrogen (NH3), and 6 mg/L dissolved oxygen (DO). DR-18-08 1 November 2018 Figure 1: Location of the proposed WWTP and WASP segmentation for the water quality model. The location of the WWTP is southwest of the intersection of US Highway 290 and Sawyer Ranch Rd. on the southern border of the Barton Creek watershed (red line). The City of Austin (COA) has an interest in maintaining the water quality of Barton Creek and the underlying Edwards Aquifer. Surface water in the contributing zone of the Edwards Aquifer, which includes Barton Creek, has previously been shown to be sensitive to nutrient enrichment (Herrington and Scoggins 2006; Mabe 2007; Herrington 2008a; Herrington 2008b; Richter 2010; Turner 2010). In aquatic systems, nutrients such as nitrogen and phosphorus support the growth of algae and aquatic plants. Nutrient enrichment can occur from the increase of either nitrogen or phosphorus to the aquatic system and can cause an increase in algal biomass to the extent that entire reaches of streams show aesthetic degradation (Wharfe et al. 1984; Biggs 1985; Biggs and Price 1987; Welch et al. 1988), loss of pollution- sensitive invertebrate taxa (Quinn and Hickey 1990), clogging of water intake structures (Biggs 1985), and degradation of dissolved oxygen and pH levels in the water column (Quinn and Gilliland 1989). The COA Watershed Protection Department (WPD) constructed a Water Quality Analysis Simulation Program (WASP) model to examine impacts immediately downstream of the proposed WWTP effluent. Total nutrients and chlorophyll a predictions from this model can be used as input into a parsimonious model (Chapra et al. 2014) to determine how far downstream impacts might be seen. METHODS The Water Quality Analysis Simulation Program (WASP) is a program maintained by the US Environmental Protection Agency (US EPA) and “general dynamic mass balance framework for modeling contaminant fate and transport in surface waters” (Ambrose and Wool 2017). WPD used an ‘Advanced Eutrophication’ model type in WASPv8.2 to simulate phytoplankton and benthic algae biomass in the unnamed tributary, detention ponds, and initial stretch of Long Branch tributary immediately downstream of the treated effluent in the proposed discharge permit. The WASP model simulated from 01 January 1999 through 31 December 2014 using a Euler solution technique, which is a typical solution technique for hydrodynamic models (Ambrose and Wool 2017). The maximum allowable timestep was set to 0.042 days (1 hour) so that predicted dissolved oxygen (DO) DR-18-08 2 November 2018 could be examined at different times of the day rather than using a daily response value. In WASPv8.2, the user defines which state variables will be incorporated into a simulation. A list of the state variables simulated can be seen in Table 1. Table 1: List of state variables used in the WASP model. WASP System Type Description WTEMP DISOX NH-34 NO3O2 ORG-N D-DIP ORG-P CBODU CBODU DET-C DET-N DET-P PHYTO MALGA MALGN MALGP Water Temperature Dissolved Oxygen Ammonia Nitrate-nitrite Organic Nitrogen Inorganic Phosphorus Organic Phosphorus Background CBOD WWTP CBOD Detritus Carbon Detritus Nitrogen Detritus Phosphorus Phytoplankton biomass MacroAlgae (Benthic) Biomass MacroAlgae (Benthic) Nitrogen MacroAlgae (Benthic) Phosphorus Segmentation of the model was created with ArcGIS coupled with site visits to procure depths of ponded segments (Table 2). Slopes were calculated by taking the difference in elevation using 0.61 m (2 ft) contours at the beginning and end of the segment and dividing that value by the length of the segment. Roughness coefficients (manning’s n) were estimated based on visual assessment of the channel (Chow 1959). The depth multiplier is the depth of the segment under average flow conditions and the depth exponent was taken from empirical hydraulic exponents that represent ephemeral streams in the semiarid US (Ambrose and Wool 2017). DR-18-08 3 November 2018 Table 2: WASP segment names, transport mode for flow, and channel geometry for each segment. Transport Length Width Slope Roughness Depth Depth Multiplier Exponent Weir Height Kinematic 90.25 1.52 0.0068 0.05 0.6096 0.36 Segment Name DS Longbranch 2 DS Longbranch 1 pond5 pond4 pond3 Between Ponds 2 and 3 pond2 pond1 US Longbranch 4 US Longbranch 3 US Longbranch 2 US Longbranch 1 DS Trib Wave Wave Ponded Weir Ponded Weir Ponded Weir Wave Ponded Weir Ponded Weir Wave Wave Wave Wave Wave Wave Wave Kinematic 153.97 1.52 0.0238 0.05 0.6096 0.36 71.54 11.7 99.67 28.93 130.45 20.38 125.84 42.37 202.69 54.10 0.3658 0.00 1.03 0.7620 0.00 2.13 1.3411 0.00 2.56 1.6154 0.00 2.23 1.7374 0.00 2.35 Kinematic 89.36 0.82 0.0478 0.025 0.1524 0.36 Kinematic 115.13 0.82 0.0265 0.05 0.3048 Kinematic 233.01 0.67 0.0209 0.04 0.3048 Kinematic 90.84 0.61 0.0201 0.02 0.1524 Kinematic 156.86 0.61 0.0039 0.02 0.0762 Kinematic 274.84 0.64 0.0270 0.03 0.0762 0.36 0.36 0.36 0.36 0.36 0.36 0.36 US Trib Kinematic 410.03 0.49 0.0178 0.015 0.0762 WWTP Kinematic 64.01 0.61 0.0476 0.02 0.0762 Time functions and parameters included in the model were solar radiation, air temperature, wind speed, light extinction, ammonia benthic flux, phosphorus benthic flux, and sediment oxygen demand. Solar radiation, air temperature (minimum and maximum), and wind speed were obtained from the National Climatic Data Center (NOAA Satellite and Information Service). Ammonia benthic flux, phosphorus benthic flux, and sediment oxygen demand were set to 0.015 mg/m2-day, 0.015 mg/m2-day, and 1.0 g/m2- day, respectively, for each WASP segment based on previous WPD WASP modeling efforts. Light extinction was set to 0.813/meter for each WASP segment based on photosynthetic photon flux data collected using a quantum meter in Onion Creek. A full list of the constants used in the model can be seen in Appendix A. Constants were taken from previous WPD WASP modeling efforts (Richter 2010; Richter 2016) with the exception of the maximum nitrogen and phosphorus uptake constants for macro algae and algal stoichiometry. These constants were set to the default values. The maximum nitrogen and phosphorus uptake constants were set to the default values because they were impairing phytoplankton growth within the system. Stoichiometry was set to default values because WPD has not obtained any biologic data from the ponds in the current model for calibration. DR-18-08 4 November 2018 Daily flows were input into WASP segments US Longbranch 1, US Longbranch 3, US Longbranch 4, Pond 1, Pond 2, Between Ponds 2 and 3, Pond 3, Pond 4, DS Longbranch 1, US Trib, and DS Trib. (Figure 1). Flows into US Longbranch 1, US Trib, and DS Trib were assumed to be headwater flows while flows into other segments were considered to be overland flow into the segment. Flow time series were constructed using the United States Geological Survey (USGS) gage 08155200 based on drainage area at the input location relative to the drainage area at the gage (Table 3). As this section of creek network is typically dry, only storm flows were input into the WASP model. If the daily flow at the gage was 50% higher than the previous days flow then input flows for that day were considered storm flow and WASP segment flows were entered into the separate model time series, otherwise the input flows were set to zero. Additionally, a daily evapotranspiration (ET) time series was constructed using the Hargreaves method and local climatological data in the Soil & Water Assessment Tool (SWAT) (Peacock 2016) and input into the WASP model to represent evapotranspiration from ponded segments. Table 3: Percent of drainage area at each flow input for the WASP model compared to the drainage area at USGS gage 08155200. WASP Input Drainage Area Percentage of gage US Longbranch 1 US Longbranch 3 US Longbranch 4 Pond 1 Pond 2 Between Ponds 2 and 3 Pond 3 Pond 4 DS Longbranch 1 US Trib DS Trib USGS gage 08155200 (km2) 0.156 0.232 0.075 0.093 0.098 0.059 0.048 0.039 0.159 0.409 0.233 232.464 0.067% 0.100% 0.032% 0.040% 0.042% 0.025% 0.021% 0.017% 0.068% 0.176% 0.100% 100% Storm loads for water quality parameters were input into WASP as boundary time series. For days in the time series when flows into a WASP segment were non-zero values, storm concentrations were set to the storm event mean concentration (EMC) for each parameter in Table 4 for that WASP segment. Otherwise, the value was set to zero. Storm concentrations were taken from other COA work where stormwater EMCs were developed from similar areas (Glick et al. 2009). Table 4: Pollutant concentrations used as boundary time series in the WASP model. Storm EMC (mg/L) Parameter Background CBOD Ammonia Nitrate-nitrite Organic Nitrogen Inorganic Phosphorus Organic Phosphorus 1.577 0.038 0.233 0.594 0.022 0.022 To model the Sawyer-Cleveland WWTP discharge, flow and loads were input into the WASP segment labeled as WWTP in Figure 1. The flow was set to a continuous 0.004 m3/s (0.092 MGD) and loads were calculated by multiplying the WWTP pollutant concentrations by the discharge and converting the loads to kg/day. WWTP pollutant concentrations were taken as a combination of the requested permit concentrations in the application and adding nitrogen and phosphorus concentrations based on BioWin DR-18-08 5 November 2018 process modeling (Table 5). Coefficients within the BioWin process model were initiated to values used in previous modeling of the City of Dripping Springs WWTP with no nitrogen limitation in the effluent (Carollo 2015) while basins within the BioWin model were determined from the Sawyer-Cleveland permit application. BioWin results did not include an effluent concentration for inorganic phosphorus so a value of 0.5 mg/L was chosen based on the initial modeling efforts regarding the City of Dripping Springs WWTP effluent (Richter 2016). Table 5: Pollutant concentrations used in the WWTP discharge load calculations for the WASP model based on BioWin process modeling. The application contained no information regarding nitrogen or phosphorus limits. Effluent Limits Proposed in Application (mg/L) WWTP Effluent Concentrations predicted by BioWin (mg/L) Parameter WWTP CBOD Ammonia Nitrate-nitrite Organic Nitrogen Inorganic Phosphorus Organic Phosphorus 10 2 4.84 1.21 17.31 2.98 0.5 3.83 RESULTS & DISCUSSION To determine the potential impacts of the proposed Sawyer-Cleveland WWTP discharge to the Long Branch Tributary and the receiving Barton Creek, results of the model from the first segment downstream of the detention ponds (WASP segment DS Longbranch 1) will be used as input into a more parsimonious model1. When the WWTP effluent is added to the WASP model, flow from the effluent slowly fills the ponded segments and eventually enters the DS Longbranch 1 segment. After such time, this segment is constantly flowing. Total nitrogen (TN) and total phosphorus (TP) concentrations are shown to be higher than the TN and TP concentrations in the WWTP effluent (Figure 2). Biologic and chemical reactions occurring within the first detention pond convert the organic phosphorus into inorganic phosphorus, a form available to be used by vegetation, which allows for even more phytoplankton or benthic algae to grow in the downstream segments. The conversion of nutrients from excess phytoplankton growth contributes to the WWTP effluent concentrations and the combination increases the TN and TP concentrations to above the WWTP effluent concentrations in the WASP segment downstream of the ponds. Benthic algae concentrations were never above 10 mg/m2 during the simulation; however, phytoplankton concentrations ranged from 120 to 140 µg/L during blooms in this segment (Figure 3). Dissolved oxygen dropped below 5 mg/L once during the simulation period but was above 6 mg/L during the majority of the simulation (Figure 4). 1 As additional stream length gets modeled, the number of inputs increases, thereby greatly increasing the complexity of the model. A more parsimonious model can aid in estimating the impacts without engendering the additional workload. DR-18-08 6 November 2018 Nutrients at WASP Segment DS Longbranch 1 40 35 30 25 20 15 10 5 / ) L g m ( t n e i r t u N l a t o T 10 9 8 7 6 5 4 3 2 1 ) 2 / m g m ( a l l l y h p o r o h C c h t n e B i 0 Jan-99 Jan-01 Jan-03 Jan-05 Jan-09 Jan-11 Jan-13 Jan-07 Date Total Nitrogen Total Phosphorus Figure 2: Total nitrogen (mg/L) and total phosphorus (mg/L) in WASP segment DS Longbranch 1, the first segment downstream of the 5 detention ponds immediately downstream of where the WWTP effluent enters Long Branch Tributary. Algal Concentrations at WASP Segment DS Longbranch 1 0 Jan-99 Jan-01 Jan-03 Jan-05 Jan-07 Jan-09 Jan-11 Jan-13 Date Benthic Algae Phytoplankton Figure 3: Benthic algae (mg/m2) and phytoplankton (µg/L) concentrations in WASP segment DS Longbranch 1, the first segment downstream of the 5 detention ponds immediately downstream of where the WWTP effluent enters Long Branch Tributary. DR-18-08 7 November 2018 160 140 120 100 80 60 40 20 0 ) L / g µ ( n o t k n a p o t y h P l Dissolved Oxygen at WASP Segment DS Longbranch 1 16 14 12 10 8 6 4 2 / ) L g m ( n e g y x O d e v o s s D l i 0 Jan-99 Jan-01 Jan-03 Jan-05 Jan-09 Jan-11 Jan-13 Jan-07 Date Figure 4: Dissolved Oxygen (mg/L) concentration in WASP segment DS Longbranch 1, the first segment downstream of the 5 detention ponds immediately downstream of where the WWTP effluent enters Long Branch Tributary. CONCLUSIONS Simulations indicate that the portion of the Long Branch Tributary downstream of the impacted detention ponds will be transformed from an ephemeral stream to a perennial stream with high concentrations of TN, TP, and phytoplankton. Modeled concentrations of phytoplankton vary by season with blooms occurring during the warmer months at concentrations around 120 to 140 µg/L which is well above the hypereutrophic threshold of 56 µg/L (Carlson and Simpson 1996). The TN and TP concentrations in this portion of the Long Branch Tributary are predicted to be similar to the WWTP effluent concentrations. Results from this model should be incorporated into a parsimonious model to determine how far downstream the nutrients and chlorophyll a concentrations remain elevated. DR-18-08 8 November 2018 References Ambrose RB, Wool TA. 2017. WASP8 Stream Transport - Model Theory and User’s Guide. Biggs BJF. 1985. Algae: a blooming nuisance in rivers. Soil Water. 21:27–31. Biggs BJF, Price GM. 1987. A survey of filamentous algal proliferations in New Zealand rivers. New Zeal J Mar Freshw Res. 21(2):175–191. [accessed 2018 Feb 23]. http://www.tandfonline.com/doi/pdf/10.1080/00288330.1987.9516214. Carlson RE, Simpson J. 1996. A Coordinator’s Guide to Volunteer Lake Monitoring Methods. North Am Lake Manag Soc.:96 pp. Carollo. 2015. Technical Memorandum No. 1 Conceptual Design Services City of Dripping Springs South Regional Wastewater Treatment Plant. Chapra SC, Flynn KF, Rutherford JC. 2014. Parsimonious model for assessing nutrient impacts on periphyton dominated streams. J Environ Eng. 140(6). Chow V Te. 1959. Open-Channel Hydraulics. Internatio. Davis HE, editor. New York, NY: McGraw-Hill Civil Engineering Series. Glick R, Zhu T, Bai B, Hubka J, Robinson R, Mahmoud S, Manning S, Moezzi A, Selucky J. 2009. Stormwater Runoff Quality and Quantity from Small Watersheds in Austin , TX : Updated through 2008. Austin, Texas. Herrington C. 2008a. Extension of an LA-QUAL (version 8 . 0) model for the proposed HCWID#1 wastewater discharge to realistic Bear Creek temperature and flow conditions. Herrington C. 2008b. Impacts of the proposed HCWCID1 wastewater discharge to Bear Creek on nutrient and DO concentrations at Barton Springs. Herrington C, Scoggins M. 2006. Potential Impacts of Hays County WCID No . 1 Proposed Wastewater Discharge on the Algae Communities of Bear Creek and Barton Springs. Mabe JA. 2007. Nutrient and Biological Conditions of Selected Small Streams in the Edwards Plateau, Central Texas, 2005 – 06, and Implications for Development of Nutrient Criteria. Scientific. Reston, VA: U.S. Geological Survey. Peacock ED. 2016. Summary of Water Balance and Discharge Evaluation for Dripping Springs TPDES Quinn JM, Gilliland BW. 1989. The Manawatu River Cleanup - Has It Worked? Trans Inst Prof Eng New Permit. Zeal Civ Eng Sect. 16(1):22–26. Quinn JM, Hickey CW. 1990. Magnitude of effects of substrate particle size, recent flooding, and catchment development on benthic invertebrates in 88 New Zealand rivers. New Zeal J Mar Freshw Res. 24(3):411–427. doi:10.1080/00288330.1990.9516433. [accessed 2018 Feb 23]. http://www.tandfonline.com/doi/abs/10.1080/00288330.1990.9516433. Richter A. 2010. Comparison of Intermittent and Continuous Discharges on Bear Creek in WASP7.3 for Richter A. 2016. WASP Model Analysis of a City of Dripping Springs Proposed Wastewater Treatment Phytoplankton and Benthic Algae. Plant Discharge to Onion Creek. Turner M. 2010. Bear Creek Receiving Water Assessment – January 2009 – March 2010. Welch EB, Jacoby JM, Horner RR, Seeley MR. 1988. Nuisance biomass levels of periphytic algae in streams. Hydrobiologia. doi:10.1007/BF00006968. Wharfe JR, Taylor KS, Montgomery HAC. 1984. The growth of cladophora glomerata in a river receiving sewage effluent. Water Res. 18(8):971–979. doi:10.1016/0043-1354(84)90247-1. [accessed 2018 Feb 23]. https://www.sciencedirect.com/science/article/pii/0043135484902471. DR-18-08 9 November 2018 APPENDIX A: List of constants used within the WASP model by constant group. Constant Group Global Inorganic Nutrient Kinetics Organic Nutrients CBOD Dissolved Oxygen Phytoplankton Value Description 0 Fresh water = 0- Marine Water = 1 30.205 Latitude- degrees -97.995 Longitude- degrees 2 0 0.13 1.08 Nitrification Rate Constant @20 degree C (1/day) Nitrification Temperature Coefficient Half Saturation Constant for Nitrification Oxygen Limit (mg O2/L) Denitrification Rate Constant @20 degree C (1/day) Denitrification Temperature Coefficient Half Saturation Constant for Denitrification Oxygen Limit (mg O2/L) 1.04 0.1 0.075 Dissolved Organic Nitrogen Mineralization Rate Constant @20 C (1/day) 0.22 1.08 1.08 0.4 0.4 1.05 1.05 0.4 0.4 7 Dissolved Organic Phosphorus Mineralization Rate Constant @20 C (1/day) Dissolved Organic Nitrogen Mineralization Temperature Coefficient Dissolved Organic Phosphorus Mineralization Temperature Coefficient CBOD Decay Rate Constant @20 C (1/day) CBOD Decay Rate Constant @20 C (1/day) CBOD Decay Rate Temperature Correction Coefficient CBOD Decay Rate Temperature Correction Coefficient CBOD Half Saturation Oxygen Limit (mg O2/L) CBOD Half Saturation Oxygen Limit (mg O2/L) Global Reaeration Rate Constant @ 20 C (1/day) 2.667 Oxygen to Carbon Stoichiometric Ratio 1 1.08 50 20 0.05 0.05 0.125 1.045 0.044 0 0 350 0.025 0.004 0.5 0.5 0.25 0.025 Phytoplankton Maximum Growth Rate Constant @20 C (1/day) Phytoplankton Growth Temperature Coefficient Phytoplankton Carbon to Chlorophyll Ratio (mg C/mg Chl) Optimal Temperature for Growth (C) Shape parameter for below optimal temperatures Shape parameter for above optimal temperatures Phytoplankton Respiration Rate Constant @20 C (1/day) Phytoplankton Respiration Temperature Coefficient Phytoplankton Death Rate Constant (Non-Zoo Predation) (1/day) Grazability (0 to 1) Nitrogen fixation option (0 no- 1=yes) Phytoplankton Optimal Light Saturation as PAR (watts/m2) Phytoplankton Half-Saturation Constant for N Uptake (mg N/L) Phytoplankton Half-Saturation Constant for P Uptake (mg P/L) Fraction of Phytoplankton Death Recycled to Detritus N Fraction of Phytoplankton Death Recycled to Detritus P Phytoplankton Nitrogen to Carbon Ratio (mg N/mg C) Phytoplankton Phosphorus to Carbon Ratio (mg P/mg C) DR-18-08 10 November 2018 Constant Group Light Macro Algae Value Description 0 0 4 0.813 Light Option (0 - light from lat-long; 1 - input diel light; 2 - input daily light- calculated diel light) Include Algal Self Shading Light Extinction in Steele (0=Yes- 1=No) Background Light Extinction Coefficient (1/m) Macro Algal Option: 1 = Floating forms (ave light) 2=Surface Algae (Top Light); 3 = submersed; 4 = benthic algae (not transported) 0.025 MacroAlgae P:C Ratio (mg P/mg C) 0.025 MacroAlgae Chl a:C Ratio (mg Chl/mg C) MacroAlgal Growth Model- 0 = Zero Order; 1 = First OrderMacroAlgal Growth Model- MacroAlgae Max Growth Rate (gD/m2-day- or 1/day) Temp Coefficient for Macro Algal Growth 1 0.4 1.05 500 Macro Algal Carrying Capacity for First Order Model (g D/m2) 0.2 1.06 0.1 1.05 0.15 Macro Algae Death Rate Constant (1/day) 1.05 Macro Algal Respiration Rate Constant (1/day) Temperature Coefficient for Macro Algal Respiration Internal Nutrient Excretion Rate Constant for Macro Algae (1/day) Temperature Coefficient for Macro Algal Nutrient Excretion Temperature Coefficient for Macro Algal Death Macro Algal Half Saturation Uptake Constant for Extracellular Nitrogen (mg N/L) Macro Algal Half Saturation Uptake Constant for Extracellular Phosphorus (mg P/L) 0.02 135 Macro Algal Light Constant for growth (langleys/day) 0.1 10 Minimum Cell Quota of Internal Nitrogen for Macro Algal Growth (mgN/gDW) Minimum Cell Quota of Internal Phosphorus for Macro Algal Growth (mgP/gDW) 10 0.5 720 Maximum Nitrogen Uptake Rate for Macro Algae (mgN/gDW-day) Maximum Phosphorus Uptake Rate for Macro Algae (mgP/gDW-day) 50 Half Saturation Uptake Constant for Macro Algal Intracellular Nitrogen (mgN/gDW) Half Saturation Uptake Constant for Macro Algal Intracellular Phosphorus (mgP/gDW) 2 2.5 MacroAlgae D:C Ratio (mg D/mg C) 0.18 MacroAlgae N:C Ratio (mg N/mg C) 2.69 MacroAlgae O2:C Production (mg O2/mg C) 0.1 Fraction of Macro Algae Recycled to Organic N Fraction of Macro Algae Recycled to Organic P 0.1 DR-18-08 11 November 2018 Parsimonious Analysis of a Proposed Wastewater Treatment Plant Discharge to the Long Branch Tributary and Barton Creek SR-19-05; March 2019 Abel Porras, P. E. City of Austin Watershed Protection Department Environmental Resource Management Division ABSTRACT The Sawyer-Cleveland Partnership is proposing to discharge treated wastewater effluent in Long Branch, a tributary of Barton Creek. In general, discharging treated wastewater in riverine systems provides an energy source for the growth of periphyton, which has the potential to change the aesthetics of the receiving stream and adversely impact aquatic species by consuming dissolved oxygen. For the special case of discharging treated wastewater in Barton Creek, the effluent has an additional harmful influence on the water quality of the underlying Edwards Aquifer. A water quality model predicting the impact of this discharge on the receiving water bodies was developed using a simplified approach. The parsimony of the model allows for quick assessment of the impact and incorporates the variability and uncertainty of the environment through different scenarios. Inputs into the model consisted of site-specific parameters, such as flow and solar radiation, as well as more general, default values, such as periphyton growth rates and periphyton death rates. These parameter values were input into the parsimonious model, including any variability and uncertainty. The result was that mesotrophic status of Barton Creek was predicted to be between 1.2 and 27.8 miles under high and low flow conditions, respectively. INTRODUCTION Barton Creek is a significant waterway in Austin, which contributes flow to Barton Springs Pool, a popular destination spot approaching almost 1,000,000 visits a year, as well as home to two species of endangered salamanders, the Barton Springs Salamander ( waterlooensis). Furthermore, Barton Creek is located in the Barton Springs contributing and recharge zone that feeds the underlying Edwards Aquifer, a sole-source aquifer to 60,000 people. The importance of Barton Creek and other streams in the Barton Springs Zone has resulted in acquisition of open space by the City resulting in over 28,000 acres of Water Quality Protection Lands and in the promulgation of specific water quality ordinances for this area. To protect these assets, the City also actively monitors the Barton Creek watershed for potential water quality impacts to Barton Creek. In 2018, the Sawyer-Cleveland Partnership applied to the Texas Commission on Environmental Quality (TCEQ) for a new Texas Pollutant Discharge Elimination System (TPDES) permit to discharge treated wastewater effluent into the Barton Creek Watershed in the Contributing Zone of the Barton Springs Segment of the Edwards Aquifer (Proposed Permit No. WQ0015594001, EPA I.D. No. TX0137863). The Eurycea sosorum) and the Austin Blind Salamander ( Eurycea SR-19-05 1 March 2019 proposed permit would authorize a discharge of treated wastewater not to exceed a daily average flow of 0.092 MGD (92,000 gallons per day). The location of the proposed wastewater treatment plant is approximately 220 m (720 ft) southwest of the intersection of US Highway 290 and Sawyer Ranch Rd. (Figure 1). The proposed discharge would travel through a series of in-line detention ponds, approximately 9770 m (6.1 miles) through the Long Branch Tributary to Barton Creek, and approximately 37,945 m (23.6 miles) through Barton Creek to the boundary of the Recharge Zone. The permit application requested treatment standards of 10 mg/L 5-day Biochemical Oxygen Demand (BOD5), 15 mg/L total suspended solids (TSS), 2 mg/L ammonia nitrogen (NH3), and 6 mg/L dissolved oxygen (DO). Richter (2018) analyzed the impact of the proposed effluent to just downstream of the series of ponds. The result from his model was effluent concentrations in Long Branch of 31.5 mg/L of Nitrogen, 6.0 mg/L of Phosphorus, and 3.5 mg/m2 of chlorophyll-a. Figure 1: Location of the proposed WWTP and WASP segmentation for the water quality model. The location of the WWTP is southwest of the intersection of US Highway 290 and Sawyer Ranch Rd. on the southern border of the Barton Creek watershed (red line). Figure obtained from Richter (2018). This report extends Richter’s analysis to Barton Creek using Chapra’s (2014) parsimonious model, which simplifies in-stream nutrient dynamics to a set of analytic equations without engendering the additional workload required by more complicated in-stream analyses. The trade-off is that some complexity in nutrient chemistry and stream heterogeneity is lost. Porras (2016) described and applied Chapra’s model to simulate the impacts of effluent on Onion Creek from the Dripping Springs Wastewater Treatment plant. The analysis in this report takes the outputs from Richter’s WASP model as inputs to Chapra’s parsimonious model. parsimonious model estimates the impact of the proposed Sawyer effluent for the remainder of Long Branch and into Barton Creek. This report documents those results. A brief primer on the theory behind the parsimonious model will be described followed by the inputs to the model and then results. Given these inputs and preliminary watershed characteristics, Chapra’s SR-19-05 2 March 2019 THEORY The underlying theory behind the analysis in this report is that cycling of nutrients in a riverine system can be explained through four mass balances. Nitrogen and phosphorus provide a supply of food for the growth of periphyton. The periphyton then respires or excretes back the nitrogen and phosphorus to create a nutrient cycle. Additionally, the death of periphyton produces organic matter in the form of organic carbon, nitrogen, and phosphorus which is not readily available for periphyton uptake. Through hydrolysis and decomposition, the organic matter is converted back to available forms of nitrogen and phosphorus. Figure 2 below illustrates this through a schematic of the nutrient cycling. Figure 2: A schematic of the nutrient cycle in a riverine system. a) along the length of the creek. The initial The mass balances derived from the schematic can be expressed as differential equations (see Porras, 2016). The differential equations can then be solved to predict the concentrations of nitrogen, phosphorus, and periphyton (represented by chlorophyll concentration of periphyton will consume the nitrogen and phosphorus, reducing the supply of food for downstream periphyton, which in turn limits further growth of the periphyton. After some length of creek, the supply of nutrients is exhausted constraining any more growth in periphyton. Denote this length the critical distance. The constrained concentration of periphyton is then transported downstream for as long as the wastewater effluent is being discharged. An average value of 36 mg/m is suggested as the threshold by which the riverine system goes from an oligotrophic to mesotrophic state (Dodds, 2006). Applying this simple model can be used to predict the trophic status of Barton Creek. MODEL INPUTS 2 of periphyton Physical Geography Figure 3 below shows the extent of the watershed contributing to the model inputs. The end of the WASP model (and start of this model) is shown as a purple dot in the figure along with the model domain as a thick polyline. The light blue polyline represents Long Branch and the darker blue line signifies Barton Creek. Differentiating between Long Branch and Barton Creek is useful because the two water bodies operate under different hydrologic regimes. Long Branch is an intermittent stream with a 6.9 mi km 2) drainage area, whereas Barton Creek continuously has flow and a 51 mi confluence with Long Branch. Thus, the model was partitioned to take into account the low flow nature of Long Branch. Outputs from Long Branch then became input for Barton Creek using flow upstream of the confluence of Long Branch with Barton Creek. 2 (132 mi2) drainage at the 2 (17.87 SR-19-05 3 March 2019 Figure 3: Areal view of the Barton Creek watershed showing the extents of the model domain as a thick polyline. The light blue polyline represents Long Branch and the darker blue line signifies Barton Creek. Dark green dot at the downstream point of Barton Creek represents USGS gage 08155200. The dark green dot at the downstream point of Barton Creek shows the location of USGS gage 08155200, which was used to ground truth flows. The drainage areas and the corresponding flows under different conditions can be seen on Figure 4 and 5, respectively below. Drainage area along Length of the Model 100 Long Branch Barton Creek ) 2 i m ( a e r A e g a n i a r D 90 80 70 60 50 40 30 20 10 0 SR-19-05 0 2 4 6 8 10 12 14 16 18 Creek length (mi) Figure 4: Drainage area of the model domain as a function of creek length 4 March 2019 Flow rate along Length of the Model Long Branch Barton Creek ) s f c ( w o l F 120 100 80 60 40 20 0 0 High Flow Low Flow 2 4 6 8 10 12 14 16 18 Model domain (mi) Figure 5: Stream flow inputs for the model as a function of creek length The observations of stream flow at USGS gage station 8155200 show that flow will be at or less than 10 cfs roughly 40% of the time1. Denote this “low flow”. Similarly, flow at the gage station greater than 100 cfs occurs about 10% of the time. This is designated as high flow. Values of flow upstream of the gage station (Figure 5) were assumed to be proportional to drainage area. Parameter Input Values The main inputs driving the model are the influent concentrations of nitrogen and phosphorus for a given rate. These values (taken from Richter, 2018) are approximately 31.5 mg/L and 6.0 mg/L, respectively, at a discharge rate of 92,000 gallons per day (0.14 cfs). The other parameter values are default values from Chapra (2012) and are displayed in the table below. Table 1: Values for Parameter in Initial Periphyton Biomass g,T, into Chapra’s model for Austin, Texas (see Porras, 2016) Parameter Cg,T k r k d Parameter Name Growth rate of periphyton Respiration and excretion rate of periphyton Death rate of periphyton Value 200 0.2 0.3 Units mg/(m2-day) 1/day 1/day Inputting the growth rate of periphyton, C results in initial values of 207 mg/m2 of periphyton biomass in the summer, when periphyton is expected to be more abundant. From this, the model inputs given in Table 2 can be used to determine estimates of downstream phosphorus and nitrogen concentrations, as well as length of eutrophication from chlorophyll-a concentrations. 1 Over a 5-year time period beginning in Dec 2013. SR-19-05 5 March 2019 Table 2: Values for Parameter in Nutrient Concentrations Parameter k sp k sn k h k d rpa rna rca rpc rnc Parameter Name Half saturation constant for available phosphorus Half saturation constant for available nitrogen hydrolysis rate of periphyton death rate of periphyton Stoichiometric coefficients for phosphorus to periphyton Stoichiometric coefficients for nitrogen to periphyton Stoichiometric coefficients for carbon to periphyton Stoichiometric coefficients for phosphorus to carbon Stoichiometric coefficients for nitrogen to carbon Value 5 20 0.05 0.3 1.2 – 1.7 7.2 0.04 2.0 – 13.3 50 – 100 Units μg/L μg/L 1/day 1/day mgP/mgA mgN/mgA gC/mgA mgP/gC mgN/gC RESULTS Predictions from the parsimonious model are depicted in Figures 6 to 9 below. Figure 6 shows that under low flow conditions, nitrogen becomes the limiting nutrient. That is, the nitrogen concentration approaches its half saturation constant of 20 µg/L before the phosphorus concentration reaches its half saturation constant of 5 µg/L. The distance that the nitrogen concentration approaches its half saturation constant is denoted as the (34.2 km) from the model origin or roughly 15.6 miles (25.1 km) downstream of the confluence of Barton Creek with Long Branch. critical distance. Under low flow conditions, the critical distance is 21.26 miles Nitrogen Concentration during Low Flow Conditions Barton Creek Long Branch ) / L g m ( n o i t a r t n e c n o C n e g o r t i N 35 30 25 20 15 10 5 0 0 2 4 6 8 10 12 14 16 18 20 22 24 26 Figure 6: Nitrogen Concentrations along the length of the stream under Low Flow Conditions Stream Length (mi) SR-19-05 6 March 2019 This implies that the wastewater discharge provides available nutrients throughout the length of the critical distance. The impact on the stream can be more clearly seen in Figure 7, which shows the concentration of chlorophyll- a as a function of distance. This figure illustrates that the chlorophyll- a concentrations are estimated to be around 200 mg/m nitrogen becomes less available along the length of the stream, its concentration is reduced to about 100 mg/m 2. Throughout the critical distance and even further, the creek can be classified as eutrophic (Dodds, 2006). 2 at the wastewater discharge point and then, as Chlorophyll a Concentration during Low Flow Conditions 250 200 150 100 50 0 ) 2 m / g m ( n o i t a r t n e c n o C a - l l y h p o r o l h C Barton Creek Long Branch C r i t i c a l D i s t a n c e 0 2 4 6 8 10 12 14 16 18 20 22 24 26 Stream Length (mi) Figure 7: Chlorophyll -a Concentrations along the length of the stream under Low Flow Conditions Under high flow conditions, phosphorus is the limiting nutrient, as it reaches it half saturation constant of 5 µg/L faster than nitrogen reaches its half saturation constant of 20 µg/L. The critical distance under high flow conditions is 8.57 mi (13.8 km) downstream from the model origin or about 2.9 miles (4.7 km) of Barton Creek will have these elevated concentrations of phosphorus. SR-19-05 7 March 2019 Phosphorus Concentration during High Flow Conditions Barton Creek Long Branch 7 6 5 4 3 2 1 0 L ) / g m ( n o i t a r t n e c n o C s u r o h p s o h P 0 2 4 6 8 10 12 14 16 18 20 22 24 26 Stream Length (mi) Figure 8: Phosphorus Concentrations along the length of the stream under High Flow Conditions The result of elevated phosphorus during high conditions is chlorophyll mg/m 2 going down to about 100 mg/m 2 at the critical distance. Figure 9 shows the concentration of chlorophyll -a with respect to distance. -a concentrations starting at 200 Chlorophyll-a Concentration during High Flow Conditions C r i t i c a l D i s t a n c e Long Branch Barton Creek 250 200 150 100 50 0 ) m2 / g m ( n o i t a r t n e c n o C a - l l y h p o r o l h C 0 2 4 6 8 10 12 14 16 18 20 22 24 26 Stream Length (mi) Figure 9: Chlorophyll-a Concentrations along the length of the stream under High Flow Conditions During high flow conditions, the effluent concentrations from Long Branch are diluted by Barton Creek upstream of the confluence. This serves to reduce the length of the critical distance in Barton Creek to about 2 miles (3.2 km) of eutrophic conditions or about 7.5 miles (12.1 km) downstream of the model origin. SR-19-05 8 March 2019 2-day). The resulting chlorophyll a concentration under this reduction ranged SENSITIVITY and UNCERTAINTY In addition to looking at different flow events, a sensitivity and uncertainty analysis was performed on the model. For the sensitivity analysis, the periphyton growth rate, C g,T, was reduced by 50% from 200 mg/(m2-day) to 100 mg/(m from a high of 80 mg/m 2 at the origin of the model down to about 50 mg/m lower chlorophyll a values are closer to modeled values in Richter (2018). However, under the low flow scenario, the model predicts that this reduction in periphyton growth rate increases the critical distance to over 40 miles (64 km). Under high flow scenario, the reduction in periphyton growth rate increases to 9.62 miles (15.5 km). Reducing the periphyton growth rate moderates the consumption rate of nutrients as well as their concentration, thus, keeping the nutrients in the water column longer and allowing for algae growth further downstream. An uncertainty analysis was also performed where values of the different parameters in the model were selected at random from a normal probability distribution. Table 3 below depicts which parameters were changed and from what normal distribution the values were selected. The model was then run 500 times with each run using different randomly generated parameters sets. Table 3: Values for Parameter in Uncertainty Analysis 2 at the critical distance. These Parameter rpa k r k h k d a 0 Mean Standard Dev 1.0 0.2 0.05 0.3 208 0.1 0.02 0.01 0.06 17 Units ugP/ugA 1/day 1/day 1/day mg/m 2 The results from the uncertainty analysis are shown in Figures 10 and 11. Figure 10 shows the range of critical distances under low flow conditions. The figure indicates that about 98% of the model runs resulted in a critical distance between 17 miles (27 km) and 33.4 miles (53 km). This is equivalent to between 11.4 and 27.8 miles (18.3 and 44.7 km) of mesotrophic status in Barton Creek downstream of Long Branch. SR-19-05 9 March 2019 Critical Distance under Low Flow y c n e u q e r F 50 40 90 80 70 60 30 20 10 0 3 2 5 2 7 2 9 2 1 3 3 3 5 3 7 3 9 3 1 4 3 4 5 4 7 4 9 4 1 5 3 5 5 5 7 5 9 5 1 6 3 6 5 6 7 6 Critical Distance (km) Figure 10: Histogram of critical distance for 500 model runs under low flow conditions e r o M SR-19-05 10 March 2019 Figure 11 shows the range of critical distances under high flow conditions. Under this scenario, 98% of the model runs produced a critical distance of between 6.84 miles (11 km) and 9.32 miles (15 km) from the model origin. This translates to between 1.2 and 3.7 miles (1.9 and 6 km) of mesotrophic status in Barton Creek downstream of Long Branch Critical Distance under High Flow y c n e u q e r F 120 100 80 60 40 20 0 0 1 1 1 1 1 2 1 2 1 3 1 3 1 4 1 4 1 5 1 5 1 6 1 6 1 7 1 7 1 8 1 8 1 9 1 9 1 0 2 Critical Distance (km) e r o M Figure 11: Histogram of critical distance for 500 model runs under high flow conditions CONCLUSION A water quality model simulating in-stream nutrient dynamics was run for a proposed wastewater discharge in Long Branch, a tributary to Barton Creek. The parsimonious nature of the model allows for the variability of the environment to be assimilated through different scenarios. The main factor influencing the impact on the stream is stream flow. Flow in Barton Creek at a downstream USGS gage was found to vary between 10 cfs and 100 cfs. Both conditions were input into the model along with uncertainty in other parameter values. The result was that mesotrophic status of Barton Creek was predicted to be between 1.2 and 27.8 miles (1.9 and 44.7 km) under high and low flow conditions, respectively. Any flow between these two conditions can be expected to yield a deleterious change in trophic status along lengths between these two values. This change in trophic status adversely impacts the recreational and aesthetic value of Barton Creek, the habitat of the residing aquatic species, and the water quality of the underlying Edwards Aquifer. SR-19-05 11 March 2019 REFERENCES Chapra, Steven C., Kyle F. Flynn, and J. Christopher Rutherford. 2014. "Parsimonious model for assessing nutrient impacts on periphyton-dominated streams." Journal of Environmental Engineering 140.6 (2014): 04014014. Dodds, WK. 2006. Eutrophication and trophic state in rivers and streams. Limnology & Oceanography, 51: pp 671-680. Porras, A. 2016. An Analytic Water Quality Model of Onion Creek examining Impacts from a Proposed Waterwater Point Source Discharge. City of Austin Watershed Protection Department. SR-17-01. Richter, A. 2018. Analysis of a Proposed Wastewater Treatment Discharge to the Long Branch Tributary of Barton Creek. City of Austin Watershed Protection Department. DR-18-08. SR-19-05 12 March 2019